ABSTRACT
Background
Magnetic nanoparticles are valued in medical applications for their distinctive properties. In this study, we synthesized magnetic nanoparticles with the aim of employing them for drug delivery.
Materials and Methods
The nanoparticles were synthesized through the co-precipitation of ferric and ferrous salts. Subsequently, these nanoparticles were incorporated into a sodium alginate-gellan gum polymeric matrix and prepared into microbeads by the ionotropic gelation method. The microbeads underwent characterization through various techniques.
Results and Discussion
In vitro release studies were conducted to assess the release of amikacin sulfate from the microbeads, and to examine the influence of crosslinking agents and polymer concentrations. Our findings suggest that the microbeads effectively control the release of amikacin sulfate.
Conclusion
Our study demonstrates the potential of magnetic nanoparticles in drug delivery, particularly through sodium alginate-gellan gum microbeads as controlled-release carriers, offering promising prospects for medical applications.
INTRODUCTION
The realm of targeted therapies, leveraging tumor-specific attributes, is rapidly advancing, with controlled-release strategies proving effective in treating disease sites. In biomedical applications, nanomaterials designed for precise drug delivery have emerged as valuable tools. Among these, magnetic particles stand out as a method capable of reaching the necessary depths for effective tumor treatment.1–4 This study aims to enhance treatment efficacy while minimizing the need for high-dose drug administration to mitigate toxic side effects. Magnetic nanoparticles find applications across scientific disciplines, encompassing physicochemical attributes, magnetic field strength, geometric characteristics, tissue penetration depth, blood flow rate, and vascular supply, all contributing to their effectiveness as drug delivery vehicles.5–8 Furthermore, the success of these delivery systems primarily depends on nanoparticle characteristics and composition, with a particular emphasis on the careful selection of polymers. Targeted drug delivery using Magnetic Nanoparticles (MNPs) holds promise for improving drug biodistribution and encapsulation efficiency of drug molecules. This approach leads to increased internalization by cancer cells compared to healthy cells and allows therapeutic agents to be administered at lower doses, reducing the toxicity associated with traditional chemotherapy.9–11
Sodium alginate (SA) and gellan gum (GG) microbeads are versatile materials extensively employed in drug delivery applications, offering controlled and sustained release of pharmaceutical compounds.12–16 Polymers, derived from natural sources, allow for precise modulation of drug release kinetics by adjusting parameters such as bead size and cross-linking methods. Moreover, the integration of magnetic nanoparticles into these microbead systems enhances their functionality.17,18 Magnetic nanoparticles, characterized by their unique physicochemical attributes, enable site-specific drug targeting through the application of external magnetic fields. This synergy between sodium alginate, gellan gum microbeads, and magnetic nanoparticles presents a promising approach to achieving both controlled drug release and targeted delivery, advancing the field of precision medicine with potential applications in various therapeutic areas.19–21 In our current study, we have synthesized magnetic nanoparticles and incorporated them into a polymeric matrix to create microbeads. These microbeads were then subjected to a comprehensive evaluation to assess their impact on release rates and swelling behavior. Our findings demonstrate that the fabricated microbeads hold substantial promise as effective carriers for drug delivery applications.
MATERIALS AND METHODS
Materials
Gellan gum, iron(II) chloride tetrahydrate, iron(III) chloride hexahydrate, NH4OH, and amikacin sulfate were obtained from Sigma-Aldrich. Sodium alginate, crosslinker-glutaraldehyde (GA), and calcium chloride were purchased from SD Fine Chemicals Ltd., (Mumbai, India).
Synthesis of nanoparticles
The procedure for synthesizing MNPs was carried out following prior documentation.5 In a 50-mL water solution, 0.3 g of iron (II) chloride tetrahydrate and 0.8 g of iron (III) chloride hexahydrate were added, and the mixture was stirred at 400 rpm for 60 min under a nitrogen environment. After this step, 30% (w/v) NH4OH (20 mL) was added and stirred at 60°C for a 60 min period. After separating the resulting black precipitate with a magnet, they were rinsed with water, left to air-dry, and stored in an airtight container for future use.
Synthesis of microbeads
Amikacin sulfate-loaded magnetic microbeads were created using a simple gelation technique. Initially, various ratios of 2% SA and GG (as specified in Table 1) were mixed and stirred up to formation of homogenous solution. Subsequently, MNPs and amikacin sulfate were added to this solution (quantities detailed in Table 1) and subjected to ultrasonication for 1 hour. The resulting solution was added drop-wise into a CaCl2 solution using a syringe, leading to the immediate formation of spherical beads. The beads were immersed in the solution for 40 min. After decantation, the resulting wet beads were collected, and any remaining drug on the surface of the beads was removed by washing three times with double-distilled water. They were then allowed to air dry overnight at room temperature.
Sample Code | SA (% w/v) | GG (% w/v) | MNPs (mg) | Drug (mg) | GA (mL) | %EE |
---|---|---|---|---|---|---|
SG1 | 50 | 50 | 50 | 100 | 1 | 72.15 |
SG2 | 60 | 40 | 50 | 100 | 1 | 74.65 |
SG3 | 70 | 30 | 50 | 100 | 1 | 75.74 |
SG4 | 50 | 50 | 50 | 75 | 1 | 70.42 |
SG5 | 50 | 50 | 50 | 50 | 1 | 67.23 |
SG6 | 50 | 50 | 50 | 100 | 2 | 69.61 |
SG7 | 50 | 50 | 50 | 100 | 4 | 66.58 |
Placebo | 50 | 50 | 0 | 0 | 1 | 00 |
All sample formulations and compositions, as well as encapsulation efficiency (%EE)
Characterization Techniques
The FTIR spectra of amikacin sulfate, MNPs, placebo, and amikacin sulfate-MNPs-loaded microbeads were obtained within the 400-4000 cm-1 range. To evaluate their crystalline characteristics, XRD analysis were performed for developed samples. This was accomplished using a wide-angle X-ray scattering diffractometer equipped with CuKα radiation (λ= 1.54060), with a scanning rate of 10°/min. The morphology of the microbeads was examined through scanning electron microscopy (SEM) conducted with a JOEL MODEL JSM 840A instrument, operating at an accelerated voltage of 20 kV. Additionally, to gain insights into the thermal properties of the developed microbeads and MNPs, scanning calorimetry (DSC) and thermogravimetric analysis (TGA) were carried out. encapsulation efficiency was evaluated based on our previous studies.22
In vitro drug release studies
To study the release pattern of the drug from the amikacin sulfate-loaded microbeads, a dissolution study was conducted using a dissolution tester (paddle model). In this study, 30 mg of drug-loaded were packed in dialysis bags and placed in 300 mL of pH 7.4 phosphate-buffered saline (PBS) at a temperature of 37°C, with the agitation rate set at 50 rpm. At predefined time intervals, 5 mL of the dissolution medium was withdrawn, subjected to analysis using a UV-Vis spectrophotometer at a wavelength of 524 nm, and subsequently replenished with fresh medium.
Release kinetics
RESULTS AND DISCUSSION
FTIR
The Figure 1 displays the FTIR spectra of gellan gum, sodium alginate, MNPs, drug, placebo, and drug-loaded microbeads (SG4 and SG7). Gellan gum exhibits peaks at 3404, 1598, 1386, and 1040 cm-1 attributed to O-H, H-O-H, C-H, and C-O stretching frequencies, respectively, and 887 cm-1 attributed to -O-SO3 stretching frequency at C-4 of galactose. In the sodium alginate spectrum, peaks are observed at 3411, 1597, 1388, 1106 cm-1 attributed to O-H, C=O, C-H, and C-O stretching frequencies, respectively. The MNPs’ FTIR spectra display peaks at 572 cm-1 (Fe-O stretching frequency), along with 1599 and 3317 cm-1 (O-H bending and stretching frequencies). The drug spectrum exhibits a broad peak at 3406 cm-1 (N-H and O-H stretching frequency). The peaks at 1589, 1384, and 1125 cm-1 attributed to C=O, C-N, and C-O stretching frequencies, respectively, and another peak at 625 cm-1 (N-H bending frequency). The placebo microbeads spectrum exhibits peaks at 3415, 1597, 1386, and 1127 cm-1 attributes to O-H, C=O, C-H, and C-O stretching frequencies, respectively. Upon comparing amikacin sulfate-loaded microbeads with the placebo, a shift in the O-H stretching frequency from 3415 to 3430 cm-1 is observed, suggesting interaction between amikacin sulfate molecules and the polymer matrix. Additionally, the C=O stretching frequency shifts from 1596 to 1610 cm-1 in amikacin sulfate-loaded microbeads, indicating interaction between drug molecules and the polymer matrix. Furthermore, a peak at 579 cm-1, attributed to the Fe-O group of MNPs, confirms successful interaction between MNPs and the polymer matrix.

Figure 1:
FTIR spectra of GG, SA, MNPs, drug (amikacin sulfate), placebo and amikacin sulfate-loaded microbeads (SG5 and SG7).
DSC
DSC analysis was performed to understand the dispersion of drug molecules in the microbeads, and the results are displayed in Figure 2. Two peaks are evident on the thermogram of SA, an endothermic peak at 82°C caused by dehydration and an exothermic peak at 289°C caused by thermal decomposition. An endothermic peak at 280°C is caused by the degradation of GG. The DSC curve of the drug shows two endothermic peaks at 255 and 307°C, whereas these peaks are not observed in drug-loaded microbeads. This confirms that the drug molecules are dispersed at the molecular level in the polymer matrix.

Figure 2:
DSC curves of SA, GG, Drug, MNPs, Placebo and SG5 (amikacin sulfate-loaded microbeads)
TGA
The thermal stability of the fabricated microbeads was evaluated using TGA, which is shown in Figure 3. Weight loss in GG was observed in two stages. As a result of dehydration, the first weight loss step occurred between 49-138°C due to adsorbed water, followed by thermal decomposition of GG at 237-325°C, and finally, weight loss at 361-589°C. A TGA curve for SA revealed three steps for weight loss. A first step involved dehydration in the range 48-148oC, a second step involved thermal decomposition between 235-327°C and a third step involved the formation of sodium carbonate residue at 331-596°C. The TGA curve for the drug showed that weight loss occurred in two steps: a minor weight loss at 75-157°C and a major weight loss at 224-383°C. Over the range of 48- 591°C, MNPs exhibited a weight loss of 6.8%, indicating that no significant weight loss occurred. At 44 and 177°C, during the thermal degradation of placebo microbeads, there is an initial 28% decrease in weight as a result of the loss of both free and bound water. We observed a weight loss of 13% in the second step between 181 and 246°C, indicating the degradation of polymer network, and a weight loss of 17% in the third step between 255 and 590°C, indicating the degradation of polymer network. SG5 drug-loaded microbeads (TGA) also showed three consecutive weight-loss steps. The first weight loss was 26% and occurred in the range 48-165°C, where the loss of water bound to the polymer matrix is attributed to the loss of water. As a result of decomposition of the polymer matrix, weight loss of 22 and 11% was observed in the second and third steps at 168-288°C and 305-589°C, respectively. Considering the TGA results, it appears that the drug-loaded microbeads have improved in thermal stability overall.
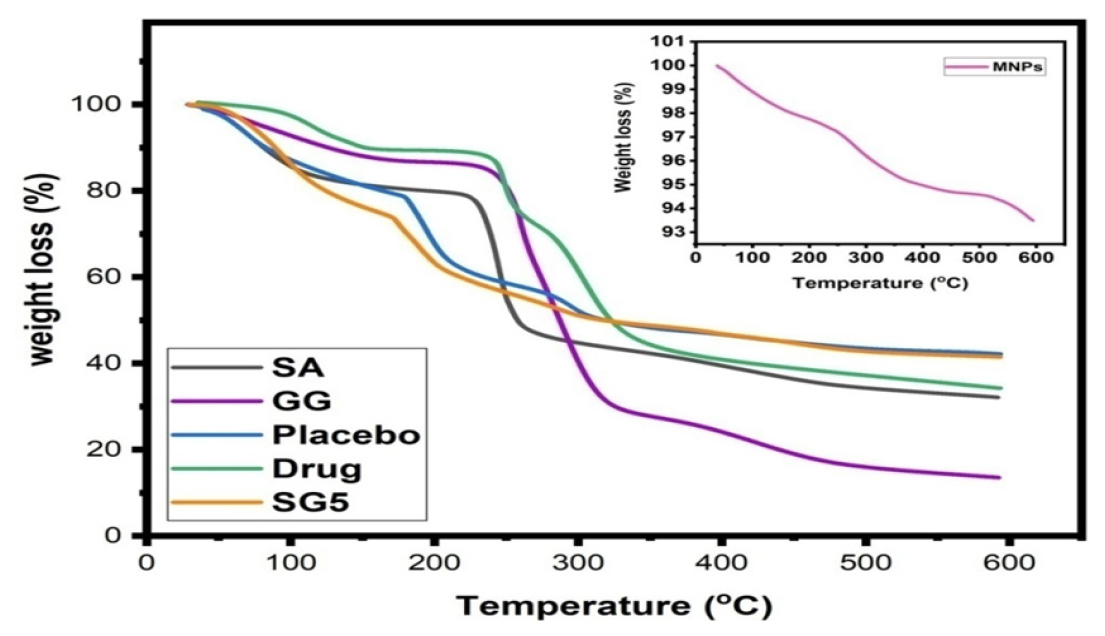
Figure 3:
A TGA curves for SG5 (drug loaded microbeads), SA, GG, and Drug.
XRD
The XRD patterns of drug, MNPs, placebo, and amikacin sulfate-loaded microbeads (SG5) are displayed in Figure 4. The generated MNPs show diffraction patterns like those of our previous results.5 The 2θ characteristic peaks of the created MNPs align with JCPDS No. 19-0629. The amikacin sulfate shows 2θ diffraction patterns between 17° and 26°, which indicates the crystalline nature of the drug. The absence of 2θ patterns corresponding to amikacin sulfate in the amikacin sulfate-loaded microbeads suggests uniform distribution of the amikacin sulfate molecules within the polymeric matrix. Moreover, the patterns associated with MNPs were identified in the drug-loaded microbeads, affirming the successful interaction between the MNPs and the polymeric matrix.
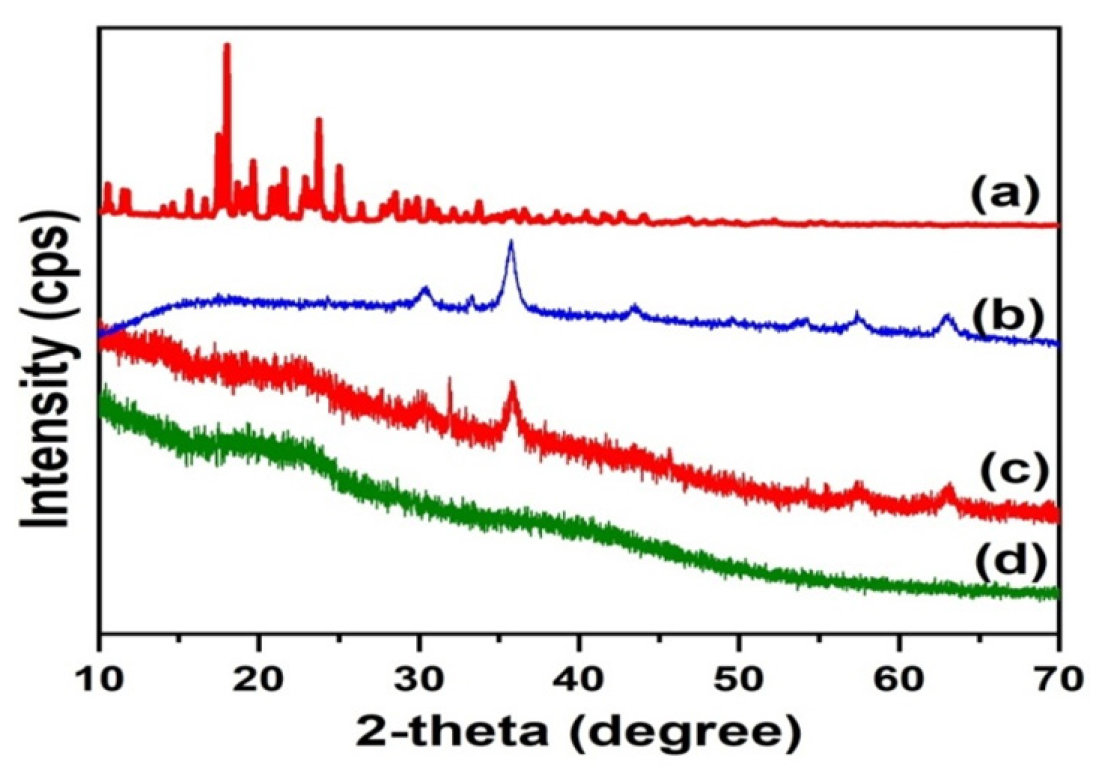
Figure 4:
XRD patterns of amikacin sulfate (a), MNPs(b), amikacin sulfate-MNPs-loaded microbeads(c) and placebo (d).
SEM
By employing SEM, an examination of the morphology of the microbeads was carried out, and the results are depicted in Figure 5. As indicated in Figure 5, it is clear that the microbeads exhibit a spherical configuration and possess a textured exterior. In particular, the outer surface of SG1 (Figures 5c and 5d) is notably more uneven compared to placebo, primarily attributed to the inclusion of nanoparticles (NPs). The typical dimensions of the produced microbeads were measured to fall within the range of 600 to 800 μm.
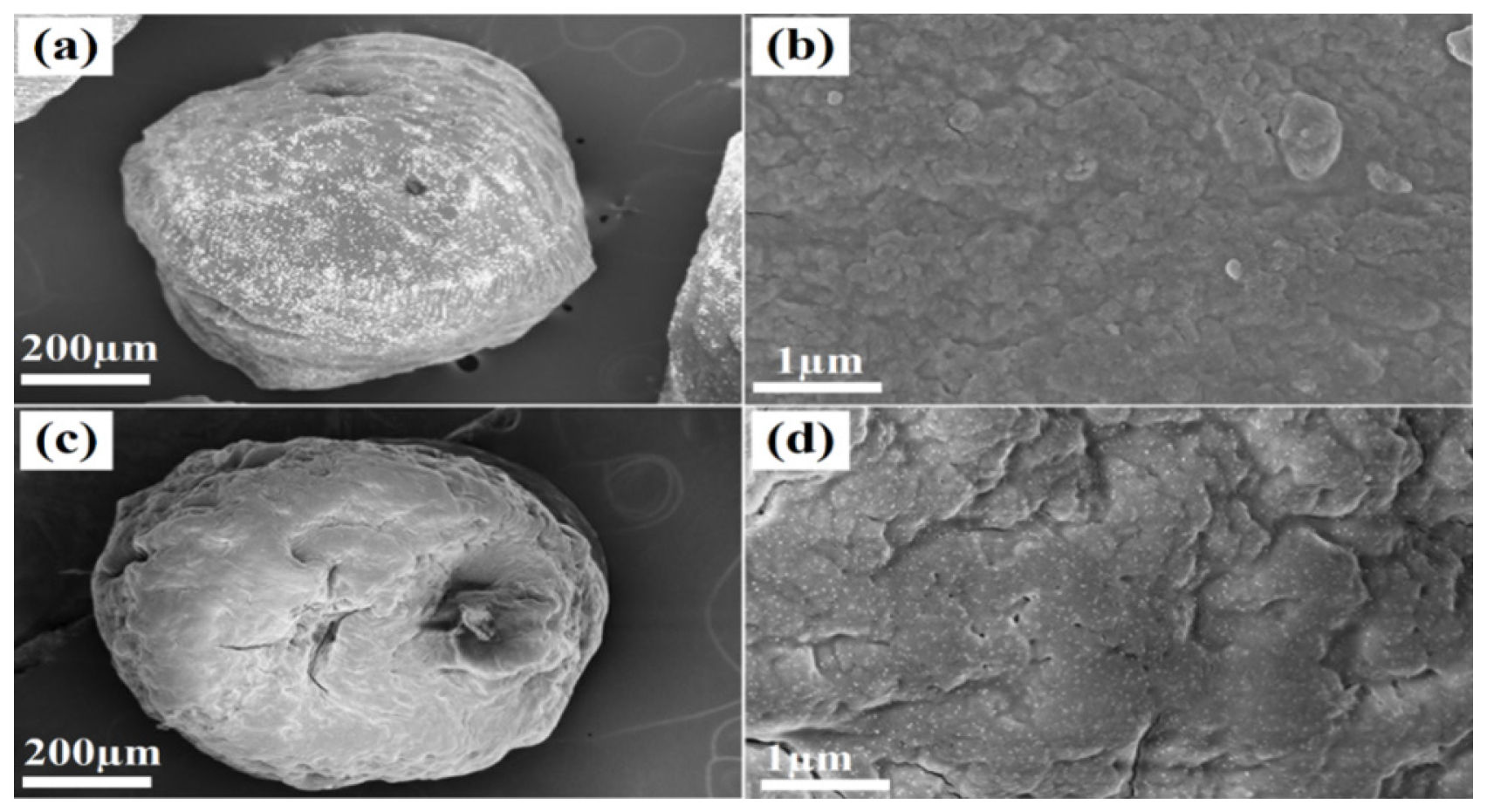
Figure 5:
Topographical images of Placebo (a and b) and amikacin sulfate-MNPs-loaded microbeads (SG1) (c and d).
In vitro drug release studies
Variation of polymers
Polymer matrix ratios were changed at constant amikacin sulfate content (50 mg) and GA content (1 mL) to control the cumulative percentage of drug release. It was apparent from Figure 6 that the overall release of amikacin sulfate escalated with a rise in the content of SA. We observe the same trend in the formulations of SG1 (50:50), SG2 (60:40), and SG3 (70:30). As a result, because SA is hydrophilic, it leaches more drug molecules into the buffer media due to its hydrophilic nature. The results of this study are consistent with those obtained by Reddy et al.22 who prepared semi-IPN microbeads made from smart karaya gum/sodium alginate.
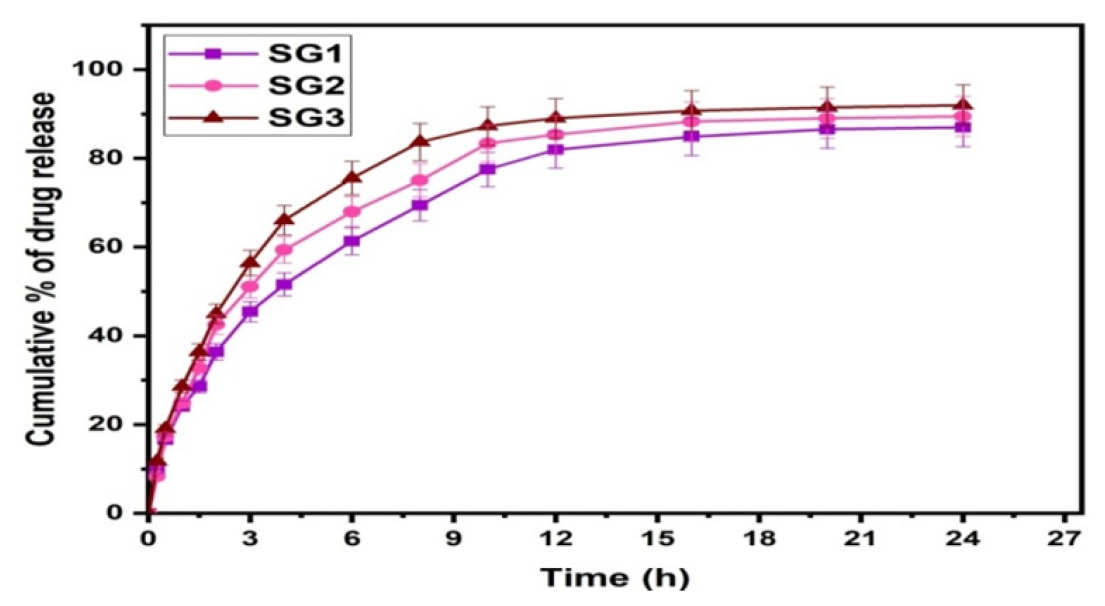
Figure 6:
Effect of polymer content on drug release.
Drug Variation
As the polymer blend ratio was held constant (50:50) and GA (1 mL), the effect of amikacin sulfate content was studied on the cumulative % of drug release. Formulations SG1 (100 mg), SG4 (75 mg), and SG5 (50 mg) were tested for the effect of drug content, and the results are presented in Figure 7. In the formulation SG1, the release rate was higher than in the formulations SG4 and SG1, resulting in the conclusion that the release rate depends on the drug content present in the matrix. There are more free void spaces in the matrix, so fewer molecules will travel through them, resulting in a lower release rate when the amount of drug is lower.
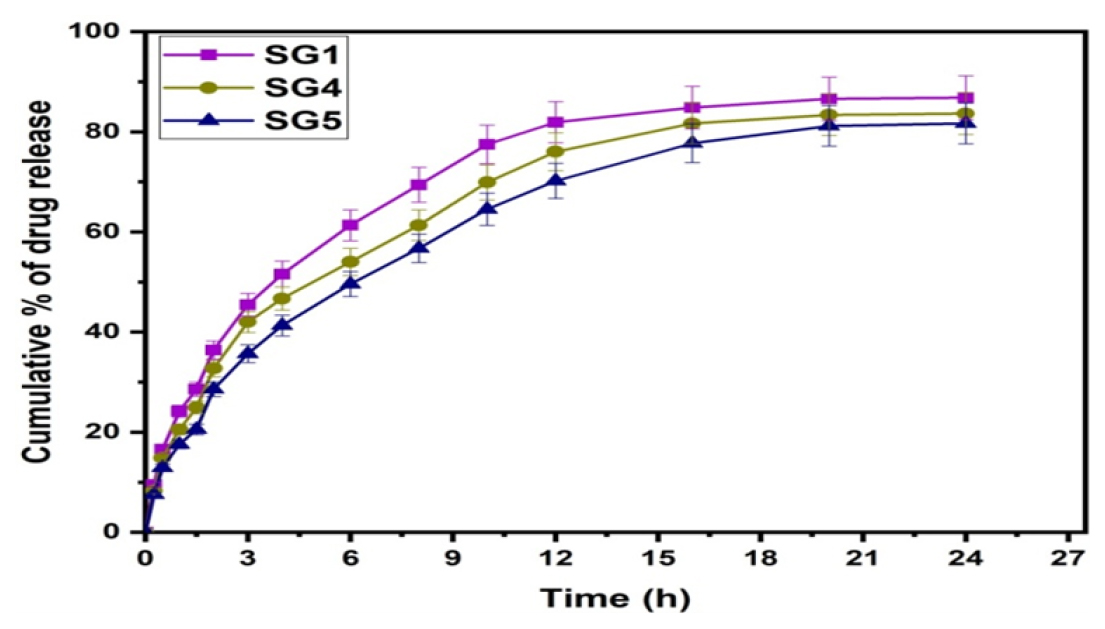
Figure 7:
Effect of drug content on drug release.
Crosslinker Variation
The formulations of SG1, SG6, and SG7 were analyzed in order to investigate the effect of crosslinker (GA) on the cumulative percentage of drug release rate shown in Figure 8. The SG1 formulation (1 mL of GA) showed a higher percentage of drug release rate than the SG6 formulation (2 mL) or the SG7 formulation (4 mL of GA), showing that as crosslinker content increased, the percentage of drug release rate decreased. Polymer networks become more rigid with an increase in crosslinker content, which reduces the chance that entrapped drug molecules can escape from the matrix and thus impedes drug release.
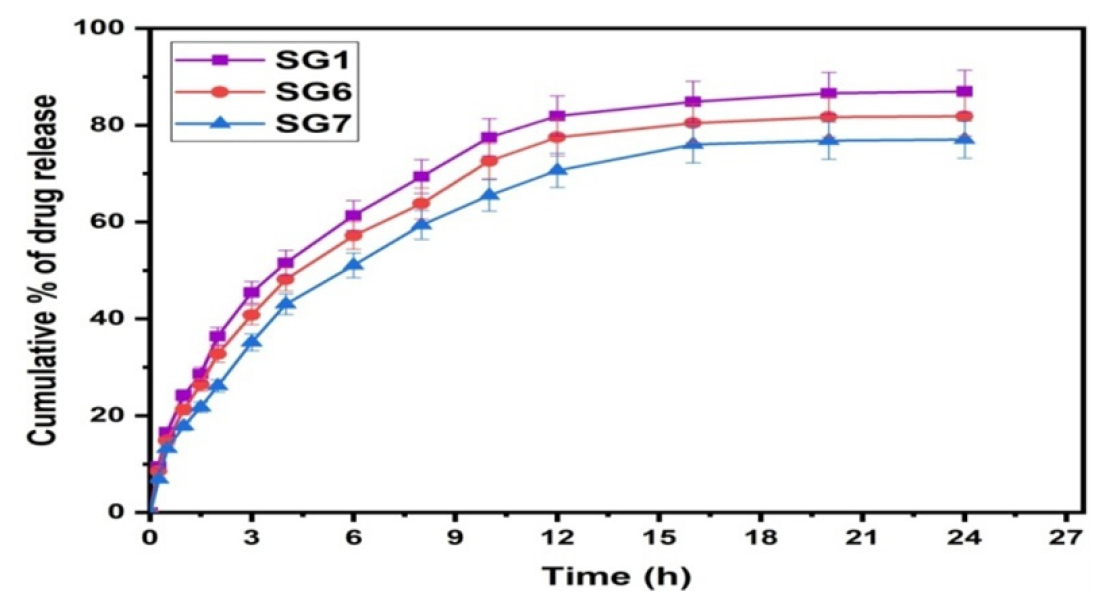
Figure 8:
Effect of crosslinker content on drug release.
Drug release kinetics
Microbeads with drug release kinetics were determined using best-fit models, including zeroth, first, and Higuchi models. As shown in Table 2, correlation coefficients (r2) for zeroth order, first order and Higuchi models are obtained from drug release kinetics. As the r2 value is close to Higuchi’s, we can assume that drug release follows Higuchi’s kinetics. A Korsmeyer-Peppas equation was fitted to the in vitro release results to confirm the mechanism.
Formulation Code | Korsmeyer-Peppas | Zero | First | Higuchi | ||||
---|---|---|---|---|---|---|---|---|
n | r2 | K0 | r2 | K1 | r2 | KH | r2 | |
SG1 | 0.564 | 0.989 | 3.195 | 0.769 | 0.0847 | 0.909 | 18.820 | 0.929 |
SG2 | 0.571 | 0.985 | 3.163 | 0.815 | 0.0755 | 0.934 | 18.370 | 0.953 |
SG3 | 0.579 | 0.990 | 3.187 | 0.856 | 0.0703 | 0.960 | 18.270 | 0.970 |
SG4 | 0.621 | 0.969 | 3.197 | 0.708 | 0.0940 | 0.881 | 19.183 | 0.890 |
SG5 | 0.571 | 0.989 | 3.138 | 0.658 | 0.1045 | 0.854 | 19.092 | 0.855 |
SG6 | 0.575 | 0.991 | 3.075 | 0.778 | 0.0712 | 0.892 | 18.064 | 0.933 |
SG7 | 0.605 | 0.989 | 3.010 | 0.805 | 0.6205 | 0.904 | 17.528 | 0.947 |
Rate constant for drug release and correlation coefficient were determined for all formulations by fitting drug release data into various mathematical models.
A drug-polymer system indicates the type of release mechanism through k, which is a constant characteristic of the drug-polymer system. We obtained values for ‘n’ ranging from 0.564-0.621, indicating the anomalous or non-Fickian diffusion type. As shown in Table 2, these results are accompanied by correlation coefficients r2.
CONCLUSION
In the present work, the magnetic nanoparticles incorporated microbeads were created by the ionic gelation technique for controlled release of amikacin sulfate. FTIR analysis verifies the successful interaction between the MNPs and the polymer matrix. The DSC results confirm that the drug molecules are dispersed at the molecular level in the matrix. XRD results revealed that the MNPs successfully interacted with the polymeric matrix. In-vitro results are fitted with the Korsmeyer-Peppas equation, and the results suggest that the created carriers followed a non-fickian diffusion type mechanism for the release of drug molecules. Analyzing the results, it was concluded that employing microbeads containing the MNPs showed significant potential as materials for drug carriers in advancing drug delivery systems.
Cite this article
Madhavi C, Ujwala G, Shahinshavali S, Jithendra T, Sreekanth OR. Fabrication and Characterization of Magnetic Nanoparticles-Embedded Polymer Blend Matrix of Sodium Alginate/Gellan Gum Beads for the Controlled Release of Amikacin Sulfate. Int. J. Pharm. Investigation. 2024;14(2):547-53.
ABBREVIATIONS
SA | Sodium alginate |
---|---|
GG | Gellan gum |
MNPs | Magnetic Nanoparticles |
GA | Glutaraldehyde |
PBS | Phosphate buffered saline |
SEM | Scanning electron microscopy |
DSC | Differential scanning calorimetry |
TGA | Thermogravimetric Analysis |
EE | Encapsulation efficiency |
XRD | X-ray diffraction |
FTIR | Fourier-transform infrared spectroscopy |
References
- Patra JK, Das G, Fraceto LF, Campos EVR, Rodriguez-Torres MDP, Acosta-Torres LS, et al. Nano based drug delivery systems: recent developments and future prospects. J Nanobiotechnology. 2018;16(1):71 [PubMed] | [CrossRef] | [Google Scholar]
- Xu M, Han X, Xiong H, Gao Y, Xu B, Zhu G, et al. Cancer nanomedicine: emerging strategies and therapeutic potentials. Molecules. 2023;28(13):5145 [PubMed] | [CrossRef] | [Google Scholar]
- Rahman MM, Islam MR, Akash S, Harun-Or-Rashid M, Ray TK, Rahaman MS, et al. Recent advancements of nanoparticles application in cancer and neurodegenerative disorders: at a glance. Biomed Pharmacother. 2022;153:113305 [PubMed] | [CrossRef] | [Google Scholar]
- Obireddy SR, Lai WF. ROS-Generating amine-functionalized magnetic nanoparticles coupled with carboxymethyl chitosan for pH-responsive release of doxorubicin. Int J Nanomedicine. 2022;17:589-601. [PubMed] | [CrossRef] | [Google Scholar]
- Obireddy SR, Chintha M, Kashayi CR, Venkata KRKS, Subbarao SMC. Gelatin-coated dual cross-linked sodium alginate/magnetite nanoparticle microbeads for controlled release of doxorubicin. ChemistrySelect. 2020;5(33):10276-84. [CrossRef] | [Google Scholar]
- McBain SC, Yiu HHP, Dobson J. Magnetic nanoparticles for gene and drug delivery. Int J Nanomedicine. 2008;3(2):169-80. [PubMed] | [CrossRef] | [Google Scholar]
- Kush P, Kumar P, Singh R, Kaushik A. Aspects of high-performance and bio-acceptable magnetic nanoparticles for biomedical application. Asian J Pharm Sci. 2021;16(6):704-37. [PubMed] | [CrossRef] | [Google Scholar]
- Laurent S, Forge D, Port M, Roch A, Robic C, Vander Elst L, et al. Magnetic iron oxide nanoparticles: synthesis, stabilization, vectorization, physicochemical characterizations, and biological applications. Chem Rev. 2008;108(6):2064-110. [PubMed] | [CrossRef] | [Google Scholar]
- Yusuf A, Almotairy ARZ, Henidi H, Alshehri OY, Aldughaim MS. Nanoparticles as Drug Delivery Systems: a review of the implication of nanoparticles’ physicochemical properties on responses in biological systems. Polymers. 2023;15(7):1596 [PubMed] | [CrossRef] | [Google Scholar]
- Ulbrich K, Holá K, Šubr V, Bakandritsos A, Tuček J, Zbořil R, et al. Targeted drug delivery with polymers and magnetic nanoparticles: covalent and noncovalent approaches, release control, and clinical studies. Chem Rev. 2016;116(9):5338-431. [PubMed] | [CrossRef] | [Google Scholar]
- Wong J, Prout J, Seifalian A. Magnetic nanoparticles: new perspectives in drug delivery. Curr Pharm Des. 2017;23(20):2908-17. [PubMed] | [CrossRef] | [Google Scholar]
- Reddy OS, Subha MCS, Jithendra T, Madhavi C, Rao KC, Mallikarjuna B, et al. Sodium alginate/gelatin microbeads-intercalated with kaolin nanoclay for emerging drug delivery in Wilson’s disease. Int J Appl Pharm. 2019;11:71-80. [CrossRef] | [Google Scholar]
- Sreekanth Reddy O, Subha MCS, Jithendra T, Madhavi C, Chowdoji Rao K. Curcumin encapsulated dual cross linked sodium alginate/montmorillonite polymeric composite beads for controlled drug delivery. J Pharm Anal. 2021;11(2):191-9. [PubMed] | [CrossRef] | [Google Scholar]
- Parandhama A, Madhavi C, Maruthi Y, Babu PK, Reddy OS, Rao KC, et al. Controlled release of verapamil hydrochloride, an antihypertensive drug from the interpenetrating blend microparticles of gelatin and gellan gum. Indian J Adv Chem Sci. 2017;5(3):176-84. [PubMed] | [CrossRef] | [Google Scholar]
- Ujwala G, Madhavi C, Reddy OS, Subha MCS, Anitha K. Synthesis and characterization of polymeric microbeads loaded with lithium cobalt oxide nanoparticles for drug delivery and antibacterial applications. J Coast Life Med. 2022;10:515-24. [PubMed] | [CrossRef] | [Google Scholar]
- Ujwala G, Madhavi C, Sreekanth Reddy O, Ramesh Raju R, Kalyankar TM, Anitha K, et al. Development and characterization of sodium alginate-g-poly(acrylic acid-c o-2-acrylamido-2-methyl-1-propane sulfonic acid)/locust bean gum microbeads containing nickel ferrite nanoparticles for pH-responsive release of doxorubicin. Mater Today Proc. 2023;92:899-905. [CrossRef] | [Google Scholar]
- Thang NH, Chien TB, Cuong DX. Polymer-based hydrogels applied in drug delivery: an overview. Gels. 2023;9(7):523 [PubMed] | [CrossRef] | [Google Scholar]
- Barbucci R, Giani G, Fedi S, Bottari S, Casolaro M. Biohydrogels with magnetic nanoparticles as crosslinker: characteristics and potential use for controlled antitumor drug-delivery. Acta Biomater. 2012;8(12):4244-52. [PubMed] | [CrossRef] | [Google Scholar]
- Fazal T, Murtaza BN, Shah M, Iqbal S, Rehman MU, Jaber F, et al. Recent developments in natural biopolymer based drug delivery systems. RSC Adv. 2023;13(33):23087-121. [PubMed] | [CrossRef] | [Google Scholar]
- Lengyel M, Kállai-Szabó N, Antal V, Laki AJ, Antal I. Microparticles, microspheres, and microcapsules for advanced drug delivery. Sci Pharm. 2019;87(3):20 [CrossRef] | [Google Scholar]
- Nguyen DT, Nguyen NM, Vu DM, Tran MD, Ta VT. On-demand release of drug from magnetic nanoparticle-loaded alginate beads. J Anal Methods Chem. 2021;2021:5576283 [PubMed] | [CrossRef] | [Google Scholar]
- Reddy OS, Subha MCS, Jithendra T, Madhavi C, Rao KC. Fabrication and characterization of smart karaya gum/sodium alginate semi-IPN microbeads for controlled release of d-penicillamine drug. Polym Polym Compos. 2021;29(3):163-75. [CrossRef] | [Google Scholar]
- Chintha M, Obireddy SR, Areti P, Marata Chinna Subbarao S, Kashayi CR, Rapoli JK, et al. Sodium alginate/locust bean gum-g-methacrylic acid IPN hydrogels for “simvastatin” drug delivery. J Dispers Sci Technol. 2020;41(14):2192-202. [CrossRef] | [Google Scholar]
- Costa P, Sousa Lobo JM. Modeling and comparison of dissolution profiles. Eur J Pharm Sci. 2001;13(2):123-33. [PubMed] | [CrossRef] | [Google Scholar]