ABSTRACT
The indole moiety, a prevalent and versatile heterocyclic scaffold, plays a pivotal role in the realm of organic synthesis, underpinning the structural basis of myriad natural products, pharmaceuticals and advanced materials. This review comprehensively surveys the landscape of methodologies for indole synthesis, highlighting the evolution of techniques from classical to contemporary strategies. We investigate the Fischer Indole Synthesis, emphasizing its historical significance and mechanistic nuances and transition to discussing the Reissert Indole Synthesis, detailing its unique contributions to the synthesis of indole derivatives. Furthermore, the review explores advanced methodologies including metal-catalyzed reactions, C-H activation processes and green synthesis approaches, offering insights into their mechanisms, scope and limitations. Through this review, we aim to provide a holistic overview of indole synthesis, furnishing researchers with a detailed understanding of its complex mechanisms and the broad utility of the indole moiety in synthesizing biologically active compounds. The synthesis methods reviewed not only underscore the chemical diversity and adaptability of indole chemistry but also highlight ongoing challenges and future directions in the synthesis and transformation of derivatives of indole. This comprehensive examination serves as a valuable resource for chemists seeking to harness the indole scaffold’s potential in novel synthetic applications.
INTRODUCTION
The indole moiety (Figure 1), characterized by a fused pyrrole and benzene ring system, stands as a cornerstone in organic chemistry due to its ubiquity in natural products and synthetic compounds. This foundational role is underscored by the indole’s participation in a variety of bioactive molecules, including alkaloids, hormones and pharmaceuticals, showcasing its significance across medicinal chemistry, material science and beyond. The indole structure’s intrinsic reactivity and versatility enable the synthesis of complex molecules, providing a scaffold for innovative drug design and development.1,2
The significance of the indole moiety extends into the realm of synthetic organic chemistry, where it serves as a critical foundational element for the structure of complex natural products and synthetic compounds. Notably, the indole nucleus’s incorporation into compounds often imparts desirable pharmacological properties, making it a target for medicinal chemistry endeavors aimed at combating various diseases.3,4 This comprehensive review aims to provide a resource for researchers in organic chemistry, aiding in the exploration of indole’s potential for future scientific breakthroughs.5
Historical Overview
Early Discoveries and Synthesis Methods
The foundation of indole chemistry can be traced back to the late 19th and early 20th centuries when initial methods focused on the rudimentary construction of the indole nucleus. One of the earliest recognized methods in indole synthesis, the Fischer indole synthesis, established in the late 1880s by Emil Fischer, provided a groundbreaking pathway for synthesizing indoles from phenylhydrazines and ketones or aldehydes under acidic conditions.9 After the Fischer synthesis, various methodologies emerged, expanding the toolbox for indole synthesis. The Leimgruber-Batcho indole synthesis (Figure 2), developed in the mid-20th century, offered an alternative for synthesizing N-substituted indoles, demonstrating the evolving complexity and efficiency of indole formation techniques.6 Moreover, the development of the Baeyer-Jackson method (Figure 3) and the application of reductive cyclization processes further diversified the approaches towards indole synthesis, enabling the creation of a vast array of indole derivatives with varying substitutions.7
Throughout the 20th century and into the 21st, the exploration of indole chemistry has continued to flourish, with newer methods such as C-H activation and functionalization directing the forefront of indole synthesis research.8 These modern approaches highlight the ongoing innovation within the field, emphasizing regioselective and environmentally conscious strategies that enhance the combination of complex derivatives of indole. Today, the synthesis of indoles encompasses a wide spectrum of methodologies, reflecting over a century of scientific inquiry and development aimed at harnessing the indole structure’s intrinsic reactivity and versatility for applications across medicinal chemistry, material science and beyond.
Evolution of Indole Synthesis Techniques
Initially, the Fischer indole synthesis served as the cornerstone for indole formation, utilizing phenylhydrazines and ketones or aldehydes under acidic conditions. This method laid the groundwork for the synthetic exploration of indoles, highlighting their potential in organic synthesis.9 In recent years, the focus has shifted towards developing more sustainable and efficient synthesis methods. Furthermore, modern synthesis techniques now employ catalytic systems and C-H activation/ functionalization-based methods, offering advancements in regioselective synthesis of functionalized indoles.8 These methods underscore the importance of indoles in pharmaceuticals, demonstrating their versatility across various fields.10 Moreover, the development of modular strategies for constructing highly strained tetracyclic structures showcases the potential for efficient and versatile synthesis of substituted indole derivatives with unique substitution profiles.
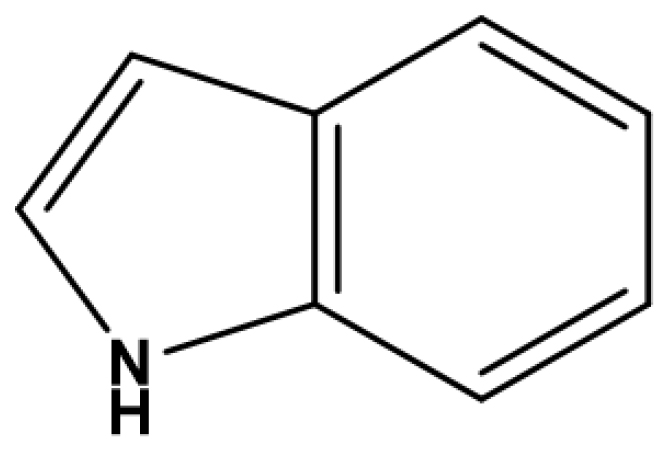
Figure 1:
Structure of indole moiety.11
Synthesis Methods
Fischer Indole Synthesis
The Fischer Indole Synthesis (Figure 4) stands as a pivotal methodology within organic chemistry, offering a straightforward route for the construction of indoles, a core structure found in many biologically active compounds and natural products. This synthesis process, named after Emil Fischer who developed it in 1883, involves the cyclization of phenylhydrazines and ketones or aldehydes under acidic conditions to yield indoles. This approach has been fundamental in the synthesis of various complex indole derivatives, showcasing its versatility and significance in synthetic organic chemistry.9,12
Over the years, the Fischer Indole Synthesis has evolved, incorporating new insights into its mechanism and extending its application through the development of novel reaction paths and conditions. Studies have focused on understanding the cyclization process in greater depth, leading to advancements in the regioselective synthesis of indoles and enhancing the efficiency and yield of these reactions.12 The introduction of three-component coupling processes and the use of environmentally friendly catalysts and solvents have further expanded the scope of this synthesis, enabling the creation of a wide array of indole derivatives with diverse functional groups and biological activities.13,14
Reissert indole synthesis
Reissert indole synthesis (Figure 5) is a well-regarded method in the domain of organic chemistry for the construction of indoles, which are crucial structures in many biologically active molecules. This synthesis technique, alongside others, has evolved significantly over the years, offering efficient routes to various indole derivatives. For instance, methods such as the Reissert-type reaction, which is catalyzed by chiral phosphoric acid, have improved the synthesis and functionalization of indoles., which affords enantioenriched indoles with strong yields and elevated enantioselectivities under mild conditions.15 Furthermore, a contemporary method involving continuous-flow hydrogenation has been developed for the synthesis of substituted indole-2-carboxylic acid ethyl esters and aza-indole analogs, demonstrating the adaptability and progression of Reissert indole synthesis in facilitating the preparation of indole derivatives efficiently.16
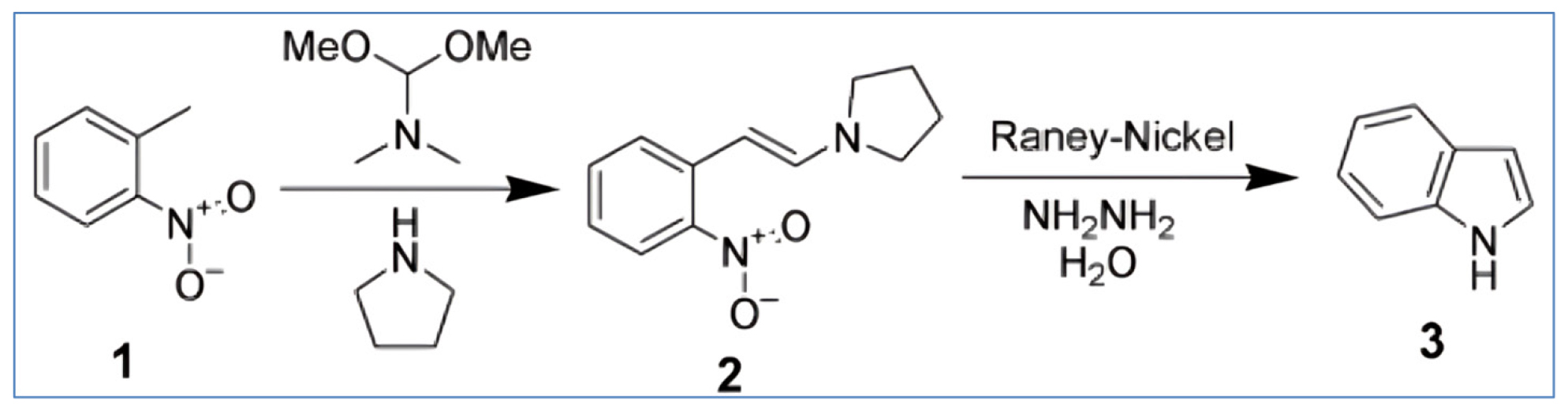
Figure 2:
Leimgruber-Batcho Indole Synthesis (mid-20th century).
Bartoli indole synthesis
Bartoli indole synthesis (Figure 6), named after its discoverer, offers a direct and versatile pathway to synthesize 7-substituted indoles, which are challenging to obtain through classical indole synthesis methods. This approach employs vinyl magnesium halides and ortho-substituted nitroarenes, showcasing its adaptability not only to heteroaromatic nitro derivatives but also to solid support applications, enhancing its utility in complex molecule construction. For instance, the synthesis on solid supports has been realized starting from Merrifield resin, leading to substituted methyl indole carboxylates with excellent purities, demonstrating the method’s compatibility with various functional groups and the potential for streamlined synthetic processes.17 Furthermore, the synthesis’s flexibility is evident in its application across different indole derivatives, including the construction of 3,3’-bis-indolylmethanes, highlighting its significant impact on organic synthesis and medicinal chemistry.18
Madelung synthesis
Madelung synthesis (Figure 7) stands for synthesizing indole derivatives, which are foundational structures in numerous natural products and pharmaceuticals. This synthesis process involves a sequence of steps starting from ortho-substituted nitrobenzenes, leveraging reductive cyclizations to forge indole cores efficiently. Notably, the process has been adapted to solid-phase synthesis, enabling the generation of 2,3-substituted indoles directly from resin-bound intermediates, showcasing the method’s versatility and applicability in modern synthetic strategies.19 Additionally, a novel approach for synthesizing 2,5-disubstituted-3-cyanoindoles demonstrates the Madelung synthesis’ adaptability and potential in accessing structurally diverse indole scaffolds, previously challenging to achieve.20
Larock indole synthesis
Larock indole synthesis (Figure 8) is an innovative method in organic chemistry for creating indole derivatives, a cornerstone structure in numerous biological compounds. Developed to address the need for a versatile synthesis approach, it uses palladium catalysis to fuse 2-iodoaniline with internal alkynes, thus producing indoles with varying substitution patterns. The process distinguishes itself by its ability to handle a wide range of functional groups, showcasing its adaptability and efficiency.21 This method opens up avenues for synthesizing complex indolic structures, such as tricyclic indoles, through intramolecular reactions, demonstrating the broad applicability of the Larock synthesis in crafting biologically relevant molecules.22
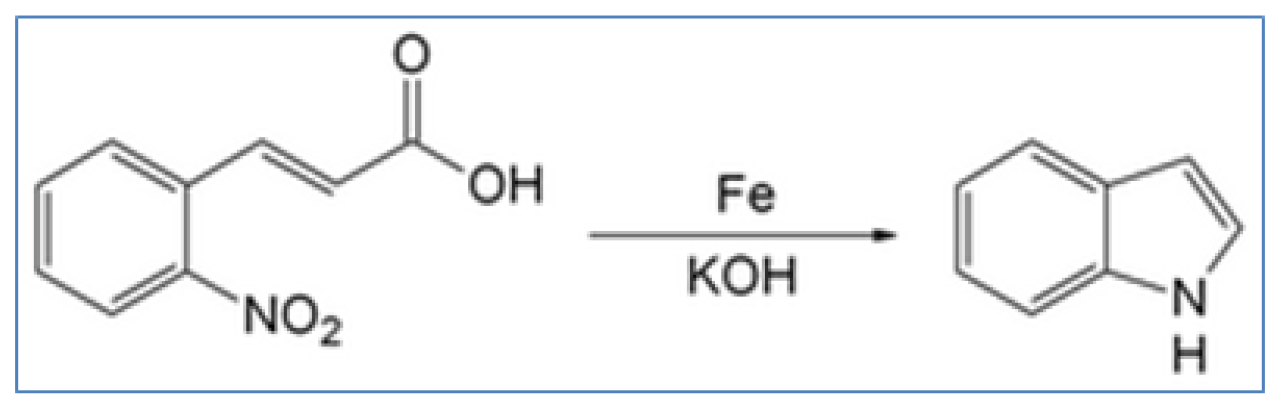
Figure 3:
Baeyer-Jackson Method and Reductive Cyclization.
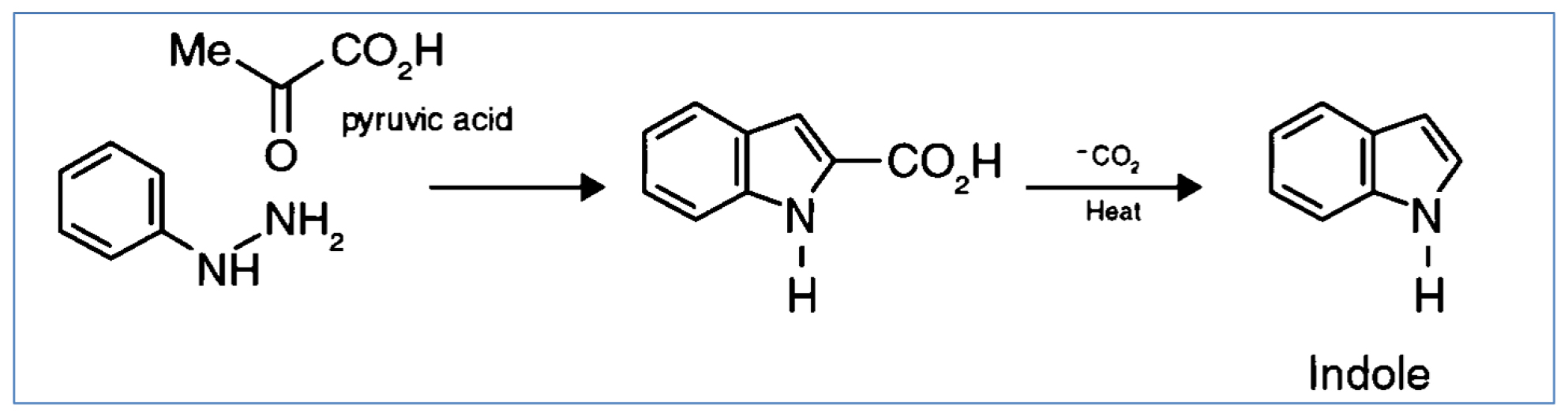
Figure 4:
Fischer Indole Synthesis (late 1880s).
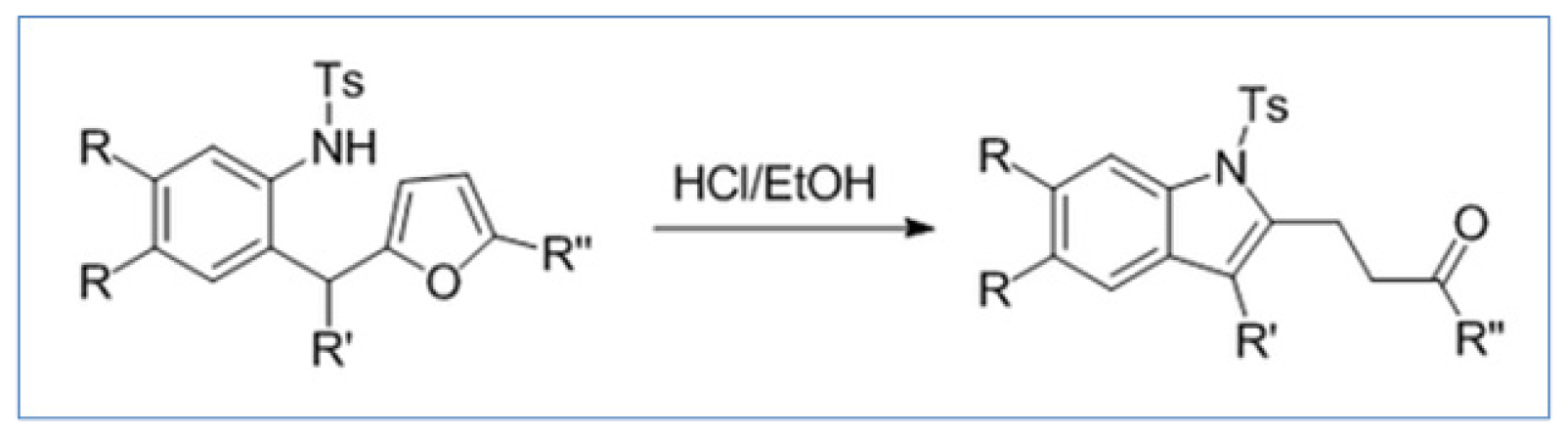
Figure 5:
Reissert indole synthesis.
Transition metal-catalyzed reactions
Transition metal-catalyzed reactions have revolutionized the synthesis of heterocycles, enabling more efficient and versatile pathways. The combination of multi-component reactions with transition metal catalysis has expanded the repertoire of heterocyclic syntheses, traditionally dominated by classical carbonyl chemistry, to include processes that utilize organometallic catalysis for both domino and sequential reactions. This integration has significantly enriched strategies for constructing diverse heterocyclic structures.23 Similarly, the addition of nitrenes and carbenes to C-H bonds, catalyzed by transition metals, has emerged as a robust method for forming C-C and C-N bonds, streamlining the synthesis of complex natural products through improved efficiency and selectivity.24
Mechanistic Insights
Mechanisms of Classical Synthesis Methods
The classical methods of mechanism synthesis have been a cornerstone in the development of complex mechanical systems, focusing on guiding movements and optimizing function through precise design. These methods, incorporating principles from kinematics and dynamics, allow for the creation of mechanisms that perform specific tasks with high efficiency. The synthesis of mechanisms, particularly for guiding or transforming motion, relies on understanding the physical principles that govern the behavior of mechanical components under various conditions. For instance, the synthesis of guidance mechanisms involves determining the optimal configuration of components to guide a point along a predetermined path, which can be achieved through the application of genetic algorithms or evolutionary techniques to find the most effective solutions.25,26
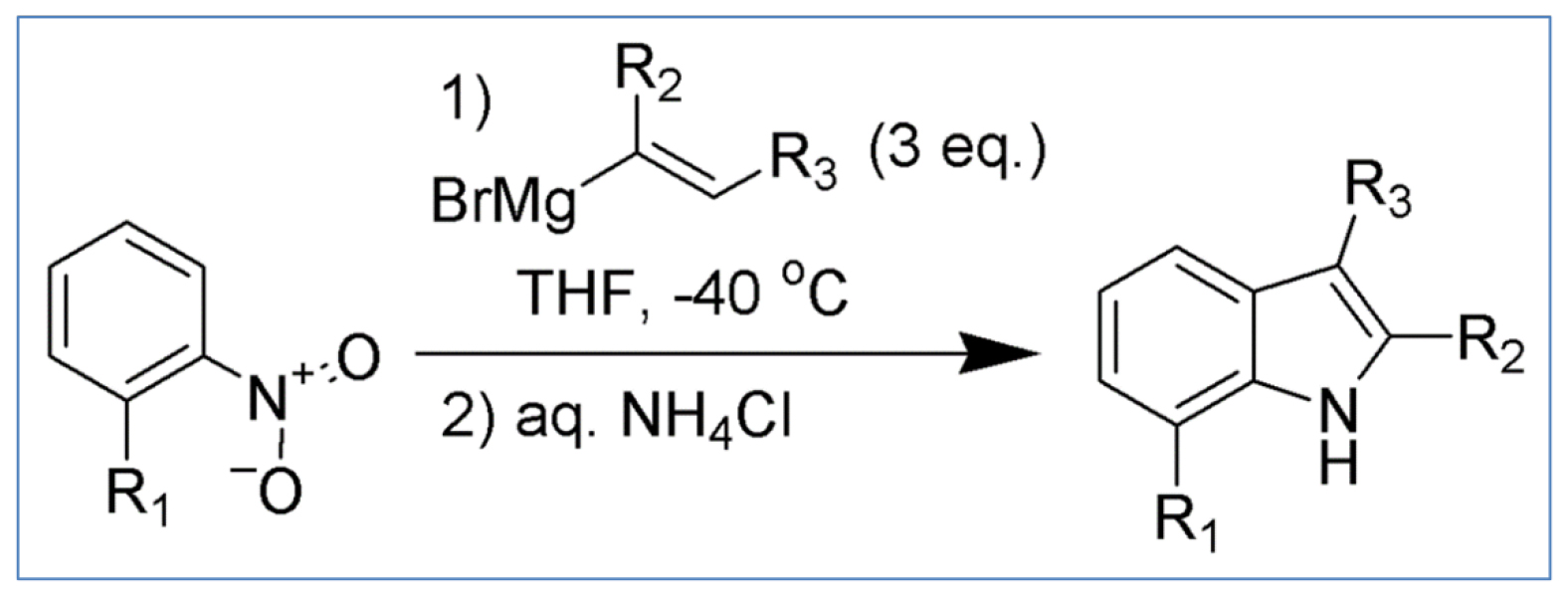
Figure 6:
Reissert indole synthesis.
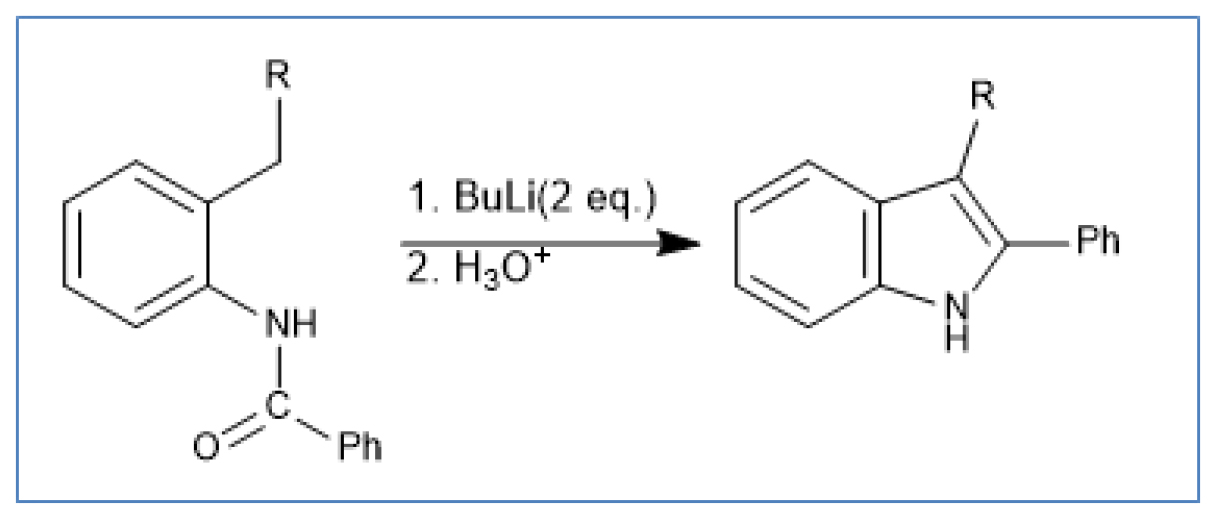
Figure 7:
Madelung synthesis.
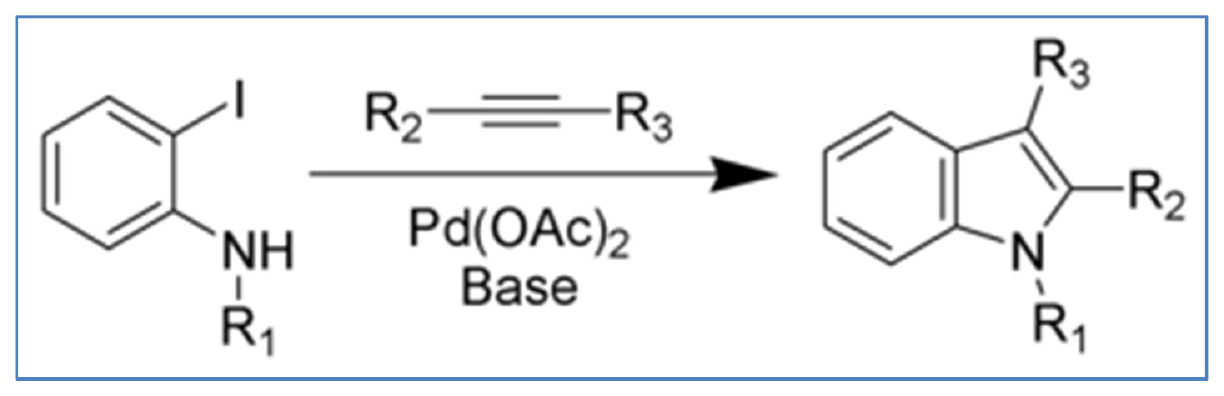
Figure 8:
Larock indole synthesis.
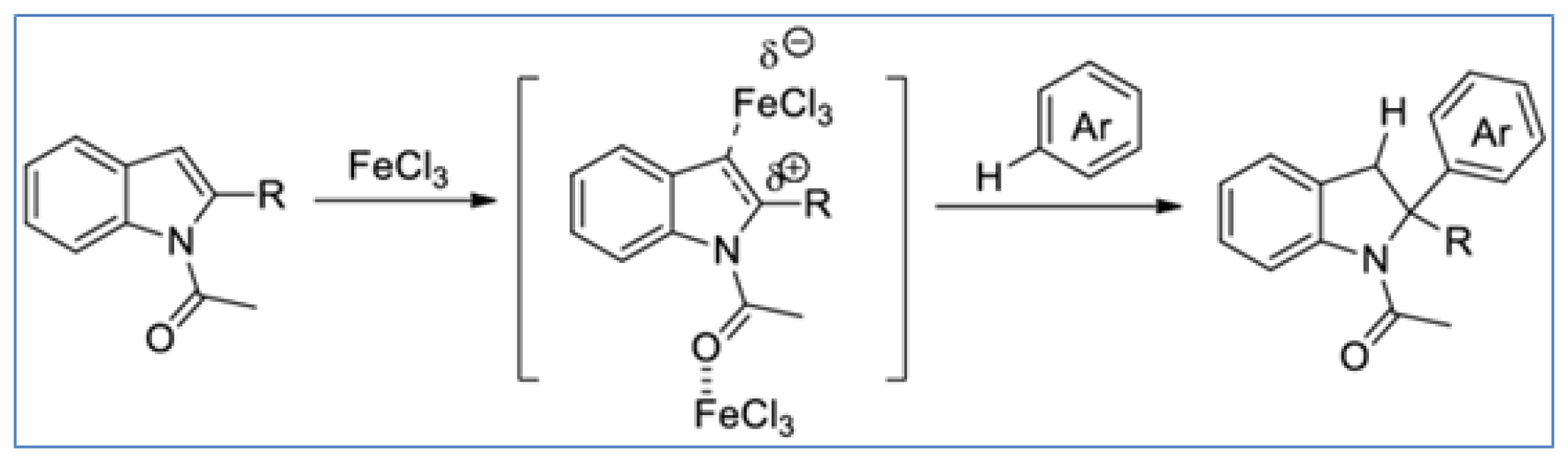
Figure 9:
C-2 Functionalization.
Catalytic Cycles in Transition Metal-Catalyzed Syntheses
Catalytic cycles in transition metal-catalyzed syntheses play a pivotal role in modern organic chemistry, enabling the transformation of simple molecules into complex structures through a series of well-orchestrated steps. These cycles often involve the formation and cleavage of bonds in a manner that is both efficient and selective. For example, Transition metal catalysis in multi-component heterocyclic synthesis has expanded the toolbox of chemists, enabling novel transformations that proceed through intricate catalytic cycles involving palladium, rhodium, ruthenium and copper catalysts.23 Moreover, transition metal-catalyzed C-H amination reactions represent another fascinating area where catalytic cycles facilitate the direct transformation of C-H bonds into C-N bonds, showcasing the power of metals such as palladium in driving these transformations through specific mechanistic pathways.27
Nucleophilic and Electrophilic Substitution Mechanisms
Nucleophilic and electrophilic substitution mechanisms are fundamental in organic chemistry, driving the transformation of molecules through the replacement of atoms or groups by nucleophiles or electrophiles, respectively. Nucleophilic substitution reactions involve a nucleophile, an electron-rich species, attacking an electrophilic center leading to the displacement of a leaving group. This mechanism is prominent in aliphatic compounds, where the type of mechanism (e.g., SN1 or SN2 ) varies based on factors like the structure of the substrate, the strength of the nucleophile and the solvent.28 Electrophilic substitution, on the other hand, typically occurs in aromatic systems where the aromatic ring acts as a nucleophile, attacking an electrophile, leading to the substitution of a hydrogen atom while preserving the aromaticity of the ring.29 These substitution reactions are not just limited to simple transformations but extend to complex organic syntheses, including the formation of heterocyclic compounds through Vicarious Nucleophilic Substitution (VNS), demonstrating their versatility and significance in synthetic chemistry.30 Understanding the stereochemistry and factors affecting these substitutions is crucial for designing efficient synthetic routes in organic synthesis.31
Rearrangements and Ring-Closure Mechanisms
Rearrangements and ring-closure mechanisms play a crucial role in organic synthesis, offering pathways to intricate structures from simpler precursors. For instance, the transition from (3Z,5Z)-octa-1,3,5,7-tetraene to (1Z,3Z,5Z)-cycloocta-1,3, 5-triene showcases a ring-closure process where the Localization function of electrons and catastrophe theory highlight the mechanistic pathways, revealing bond formation and electron pair rearrangements.32 Similarly, Ring-Arrangement Metathesis (RRM) emerges as a powerful synthetic method, combining metathesis transformations into a domino process for constructing complex structures through the interplay of ring-opening and ring-closing metathesis.33
Functionalization of the Indole Ring
C-2 and C-3 Functionalization
Functionalization of the indole ring at the C-2 and C-3 positions is pivotal in the synthesis of complex molecular structures. The selective functionalization of these positions enables the creation of several different indole derivatives, thereby expanding the utility of indoles in medicinal chemistry. For C-2 functionalization (Figure 9), a noteworthy method involves the Fe (II)-catalyzed amination of aromatic C-H bonds via ring opening of 2H-azirines, leading to the synthesis of 2,3-disubstituted indoles.34 Similarly, Two-vinylated indoles are produced when indole-3-carboxylic acids are oxidatively vinylated with alkenes by palladium-catalyzed directed C-H functionalization and decarboxylation.35 Another innovative approach includes the rhodium-catalyzed technique that demonstrates the wider range of functionalization beyond C-2 and C-3 sites for direct C-H functionalization at the C4 position of unprotected indoles.36
For C-3 functionalization, cobalt(III)-catalyzed site-selective C-H alkynylation using hypervalent iodine-alkyne reagents exemplifies the diverse functional group tolerance and provides an efficient procedure for creating C-2 alkynylated indoles.37 Additionally, a Ru (II)-catalyzed C-H activation strategy allows for the addition of maleimide selectively to indole at the C-2 location, further demonstrating the ability to selectively target indole’s reactive sites.38
N-1 Functionalization
N-1 functionalization of indoles (Figure 10) represents a significant area in synthetic chemistry, enabling the creation of diverse indole derivatives with varied biological activities. This process involves the modification of the indole scaffold at the nitrogen atom, which can significantly alter the chemical and pharmacological properties of indole-based compounds.
For instance, palladium-catalyzed reactions have become indispensable tools for the synthesis and functionalization of indoles, offering routes to a wide array of biologically active compounds. The versatility of palladium catalysis, tolerant of various functionalities, makes it ideal for complex molecule synthesis, including indole derivatization at the N-1 position.39 Similarly, N-alkoxycarbamoyl indoles’ functionalization via transition metal catalysis has been explored, with N-methoxy/ ethoxy/pivaloxy amide groups serving as effective directing groups for site-selective inert C-H functionalization, thereby enabling the synthesis of polycyclic indole frameworks.40
Moreover, the creation of metal-free conditions for the functionalization of indoles, such as the bromo-amination via 1,3-migration of imides on indolyl(phenyl)iodonium imides, underscores the growing interest in employing hypervalent iodine(III) for regioselective dual functionalization of C(sp2)-H.41 Additionally, the PIDA-driven oxidation C-C bond formation from N-aryl enamines offers a straightforward approach to synthesizing functionalized indoles, demonstrating excellent resistance to functional groups and low reaction temperatures.42
Biocatalysis also provides a fresh avenue for the C-H functionalization directly of unprotected indoles, enabling the synthesis of C3-functionalized derivatives. This approach, utilizing engineered variants of myoglobin, bypasses the limitations of traditional metal-catalyzed carbene transfer, which typically requires N-protected indoles.43
Selective Halogenation, Nitration and Sulfonation
For selective halogenation (Figure 11), a controlled α-mono- and α,α-di-halogenation of alkyl sulfones can be attained by employing Electrophilic Halogenation with Base Mediation, highlighting the importance of solvent-electrophile interactions for product control.44 Similarly, the palladium-catalyzed oxidative C-H halogenation of S-unprotected sulfides, directed by pyridinyl groups, prevents sulfur oxidation while forming C-X bonds, demonstrating a method for synthesizing sulfanyl polyhalides and polyaromatics with high selectivity.45
In the realm of nitration, the halogen-directing effects in the sulfonation of halogens-toluenes reveal that halogen substituents predominantly direct the nitration to the para position over the methyl substituent, showcasing the subtle interplay of electronic effects in determining nitration patterns.46
For sulfonation, the direct sulfonation of aromatic hydrocarbons and their halogen derivatives using various sulfur reagents is a more straightforward approach than indirect methods. This method is pivotal for introducing sulfonic acid, sulfonyl chloride and sulfonyl fluoride groups into aromatic systems, showing broad applicability and convenience.47
Applications in Organic Synthesis
Natural Product Synthesis
The indole moiety, a prominent and structurally significant component, plays a crucial role in the process of creating natural items, particularly alkaloids. This two-cycle arrangement, composed of a benzene ring fused to a pyrrole ring, is foundational in a myriad of bioactive compounds and pharmaceuticals. One of the most classical and still relevant methods for synthesizing indoles is the Fischer Indole Synthesis (FIS), which has been widely utilized throughout the whole process of creating natural products. This method illustrates the enduring value of FIS in generating indole rings that form the core of many alkaloids and other biologically active compounds.48
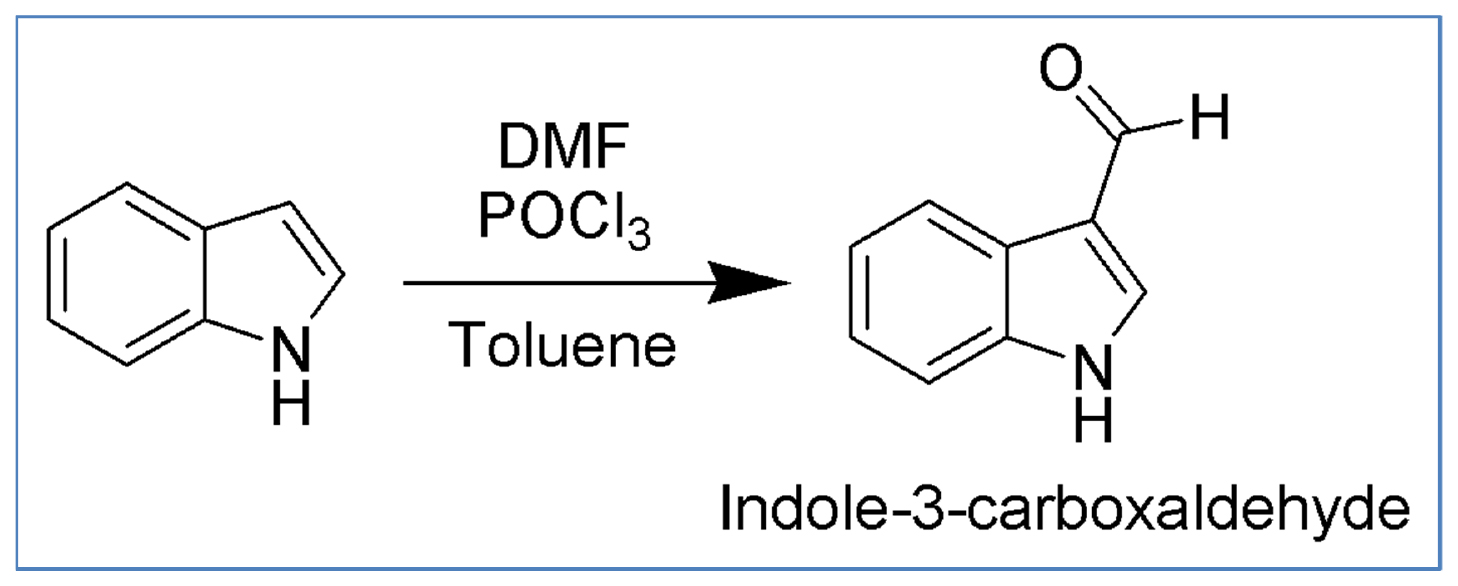
Figure 10:
N-1 Functionalization (Vilsmeier-Haack reaction with indole).
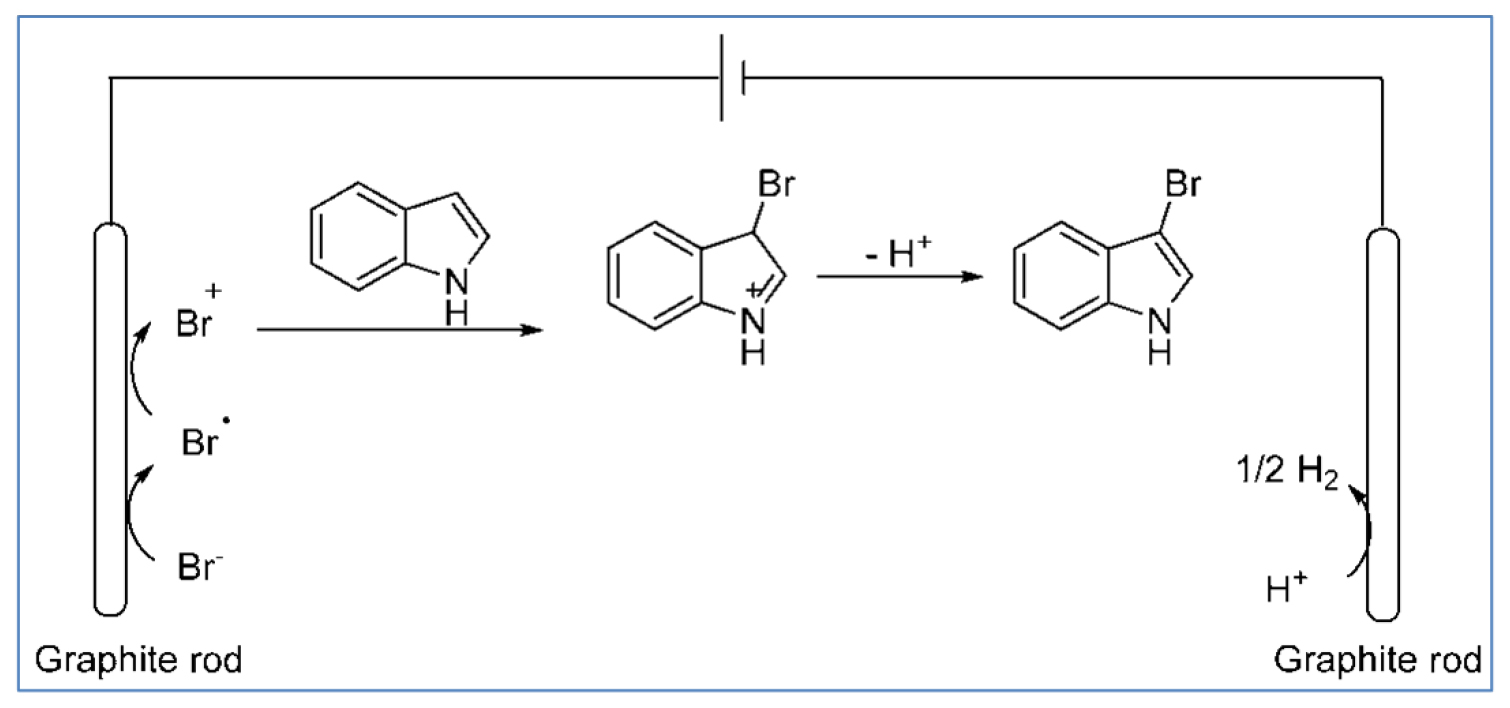
Figure 11:
Selective Halogenation, Nitration and Sulfonation.
Furthermore, palladium-catalyzed reactions have become an effective synthesis technique. and functionalization of indoles. These methods have been instrumental in constructing the indole nucleus of many natural products, demonstrating the critical Palladium’s involvement in catalysis in the modern synthesis of complex molecules.49 Recent advances have also showcased the synthesis of indole derivatives through green approaches, emphasizing the importance of sustainable methodologies in the construction of indole-based compounds. These methods highlight the ongoing innovations in indole synthesis, contributing to the development of pharmaceuticals and the exploration of new biological activities.50 Indoles are also key scaffolds in multicomponent reactions, a testament to their utility in creating diverse heterocyclic compounds. This approach has facilitated the design of novel polycyclic structures by incorporating multiple fused heterocyclic scaffolds, thereby expanding the chemical and biomedical relevance of indole derivatives.51
Pharmaceutical Applications
Indole derivatives hold a pivotal position in pharmaceutical chemistry because they engage in a variety of biological activities, making them essential components in the development of therapeutic agents. The versatility of the indole moiety allows for its incorporation into a myriad of pharmacologically active molecules targeting various diseases, including cancer, viral infections, psychiatric disorders, hypertension, migraines, arthritis and pain management.
New advances in the production of derivatives of indole through green methodologies have further enhanced their pharmaceutical applicability. These green approaches offer advantages such as high yields, short reaction times, cost-effectiveness, efficiency and environmental friendliness, addressing the limitations of conventional synthetic methods. The shift towards green chemistry underscores the commitment to sustainable practices in drug development and highlights the ongoing innovations in the synthesis of indole-based pharmaceuticals.50
Indole derivatives exhibit a broad spectrum of biological activity, such as antioxidant, antibacterial, antitubercular, antiviral, anti-inflammatory, anticancer, anti-HIV, antidiabetic, antimalarial and anticholinesterase properties. This wide range of activities has fueled research into synthesizing a variety of indole derivatives, driven by their significant therapeutic potential and the pursuit of novel treatments for diverse medical conditions.52
The importance of indole derivatives in the pharmaceutical landscape is further highlighted by their role in Central Nervous System (CNS) disorders. Several marketed drugs for CNS disorders, such as binedaline, amedalin, pindolol, siramesine and oxypertine, feature the indole moiety. This focus on CNS-active indole derivatives emphasizes their critical role in addressing mental health conditions and neurological disorders, providing a foundation for future drug development efforts targeting the CNS.53
Moreover, the medicinal significance of indoles is evident in the Structure-Activity Relationship (SAR) studies of various indole analogs, which have led to the discovery of new drug candidates to address pharmacological conditions. Indole-derived compounds like vincristine (anticancer), reserpine (antihypertensive) and amedalin (antidepressant) exemplify the medicinal and pharmacological importance of the indole nucleus in improving human health.54
DISCUSSION
The indole moiety, with its distinct bicyclic structure made out of a fused benzene ring to a pyrrole ring, occupies a venerable position in the realm of organic synthesis. Its ubiquity in a vast array of natural products, pharmaceuticals and materials science underscores its importance. Recent advances in indole chemistry have significantly expanded the toolbox for synthetic chemists, enabling the construction of complex indole architectures with unprecedented efficiency and selectivity. This discussion delves into these advancements, spotlighting novel methodologies, mechanistic insights and the burgeoning application of indoles in drug synthesis.55
Recent developments in Multicomponent Reactions (MCRs) have unveiled new vistas for the synthesis of indole derivatives, illuminating the path toward complex heterocyclic structures with significant biological and pharmaceutical potential. The integration of indoles in MCRs allows for the design of polycyclic molecules by incorporating multiple fused heterocyclic scaffolds, aiming to yield new compounds of chemical and biomedical relevance.51 Furthermore, the exploration of new synthetic pathways, including contemporary iterations of traditional syntheses, such as the Fischer, Nenitzescu and Reissert syntheses, alongside novel approaches involving heterocycle conversions, synthesis from o-alkynyl anilines and N-heterocyclic carbene catalysis, reflects the dynamic nature of indole synthesis research.58 The advent of palladium-catalyzed reactions has marked a significant milestone in indole chemistry, offering a flexible platform for the synthesis and functionalization of indoles. These methodologies, known for their wide tolerance of functionalities, facilitate the elaboration of complex molecules, thereby revolutionizing traditional synthetic strategies and significantly reducing waste.49 Additionally, the utilization of chemistry of flow in the synthesis of indole derivatives exemplifies the shift towards more sustainable and efficient processes. Flow technology, by enhancing reaction rates and selectivities, has become an effective instrument. in the synthesis of pharmaceutically relevant indoles.56
The synthesis of indoles catalyzed by transition metals such as copper, ruthenium, rhodium, palladium, or gold has been extensively explored, demonstrating the versatility and efficiency of these catalytic systems.57 Recent advancements also underscore the role of cascade reactions in the synthesis of indoles and quinolines, offering streamlined approaches to these heterocycles via several bond-forming and bond-cleaving processes carried out in a single synthetic process.58
The regioselective synthesis of indoles via C-H activation/ functionalization has become a focal point of recent research, highlighting the strategic synthesis of 2-substituted, 3-substituted and 2,3-disubstituted indoles. This progress reflects a broader trend toward the development of methodologies that enable precise manipulation of the indole scaffold, facilitating the creation of structurally diverse compounds.8
CONCLUSION
In conclusion, the indole moiety stands as a fundamental and versatile heterocyclic scaffold in organic synthesis, serving as the cornerstone for numerous natural products, pharmaceuticals and advanced materials. This review has thoroughly explored methodologies for indole synthesis, tracing the evolution of techniques from classical to modern strategies. The discussion encompassed the historical significance and mechanistic intricacies of the Fischer Indole Synthesis and the unique contributions of the Reissert Indole Synthesis to indole derivative synthesis. Moreover, advanced methodologies such as metal-catalyzed reactions, C-H activation processes and green synthesis approaches were examined, shedding light on their mechanisms, applicability and limitations. By offering a comprehensive overview, this review aims to equip researchers with a profound comprehension of the complex mechanisms underlying indole synthesis and the broad utility of indole moiety in creating biologically active compounds.
Cite this article:
Porwal A, Rajendiran A, Alam P, Singh H, Singh K, Dubey A. Indole Moiety in Organic Synthesis: A Comprehensive Review of Methods and Mechanisms. Int. J. Pharm. Investigation. 2024;14(4):1052-60.
ACKNOWLEDGEMENT
We sincerely acknowledge the Honorable Vice-Chancellor of CSJM University, Kanpur for the resources and substantial support.
ABBREVIATIONS
THF | Tetrahydrofuran |
---|---|
BuLi | Butyllithium |
H3O+ | Hydronium ion |
Pd(OAc)2 | Palladium(II)acetate |
VNS | VicariousNucleophilic Substitution |
RRM | Ring-Rearrangement Metathesis |
FeCl3 | Iron (III) chloride |
DMF | Dimethylformamide |
POCl3 | Phosphoryl chloride |
PIDA | Phenyliodine(III)diacetate |
FIS | Fischer Indole Synthesis |
SAR | Structure-Activity Relationship |
MCRs | Multicomponent Reactions |
References
- Ghosal A. Application of Palladium Chemistry in Organic Synthesis Techniques: the Larock Indole Synthesis, a Literature Review. Vertices. 2023;1(2):1 [journal] [CrossRef] | [Google Scholar]
- Henary M, Kananda C, Rotolo L, Savino B, Owens EA, Cravotto G, et al. Benefits and applications of microwave-assisted synthesis of nitrogen-containing heterocycles in medicinal chemistry. RSC Adv. 2020;10(24):14170-97. [PubMed] | [CrossRef] | [Google Scholar]
- Kaur J, Utreja D, Ekta EN, Jain N, Sharma S. Recent developments in the synthesis and antimicrobial activity of indole and its derivatives. Curr Org Synth. 2019;16(1):17-37. [PubMed] | [CrossRef] | [Google Scholar]
- Snieckus V, Gupta S. Reductive Fischer indole synthesis from N-aryl conjugated hydrazones. Chem Eur J. 2016;22:2616-9. [CrossRef] | [Google Scholar]
- Valentin Mercado-Marin E. n.d. [Book]. Indole alkaloids an introduction to the enamine chemistry of natural products The Commonwealth & International Library of science technology in organic chemistry advanced section. [CrossRef] | [Google Scholar]
- Vetelino MG, Coe J, Bradlee MJ. Convenient preparation of N-substituted indoles by modified Leimgruber-Batcho indole synthesis. 2010;37:6045-604. [CrossRef] | [Google Scholar]
- Gribble GW. Indole ring synthesis: from natural products to drug discovery. 2016:363-80. [CrossRef] | [Google Scholar]
- Kumar I, Kumar R, Sharma U. Recent advances in the regioselective synthesis of indoles via C-H activation/functionalization. Synthesis. Synthesis. 2018;50(14):2655-77. [CrossRef] | [Google Scholar]
- Przheval’skii NM, Kostromina LY, Grandberg II. New data on the mechanism of the Fischer indole synthesis (review). Chem Heterocycl Compd. 1988;24(7):709-21. [CrossRef] | [Google Scholar]
- Patel PC, Pareek A, Kishore D. Synthesis and evaluation of analogues of indole. Asia Jour Rese Chem. 2016;9(7):296-328. [CrossRef] | [Google Scholar]
- Smith AB, Kürti L, Davulcu AH. A new modular indole synthesis. Construction of the highly strained CDEF parent tetracycle of Nodulisporic acids A and B. Org Lett. 2006;8(10):2167-70. [PubMed] | [CrossRef] | [Google Scholar]
- Raffaello F, Franco S. Studies on the Fischer indole synthesis part 2. Chem Informationsdienst. 1975;6(4):813-7. [CrossRef] | [Google Scholar]
- Ghiyasabadi Z, Bahadorikhalili S, Saeedi M, Niyazagheh KM, Mirfazli SS. SBA-15-Pr-SO 3 H catalyzed one-pot synthesis of indole derivatives via Fischer indole pathway. J Heterocycl Chem. 2019:1-5. [CrossRef] | [Google Scholar]
- Kaim EL, Grimaud L, Ronsseray C. Three-component Fischer indole synthesis. Chem Inform. 2011;15:2296-8. [CrossRef] | [Google Scholar]
- Cai Y, Gu Q, You SL. Chemoselective N-H functionalization of indole derivatives via the Reissert-type reaction catalyzed by a chiral phosphoric acid. Org Biomol Chem. 2018;16(33):6146-54. [PubMed] | [CrossRef] | [Google Scholar]
- Colombo E, Ratel P, Mounier L, Guillier F. Reissert indole synthesis using continuous-flow hydrogenation. J Flow Chem. 2011;1(2):68-73. [CrossRef] | [Google Scholar]
- Knepper K, Bräse S. Bartoli indole synthesis on solid supports. Org Lett. 2003;5(16):2829-32. [PubMed] | [CrossRef] | [Google Scholar]
- Abe T, Nakamura S, Yanada R, Choshi T, Hibino S, Ishikura M, et al. One-pot construction of 3,3’-bisindolylmethanes through Bartoli indole synthesis. Org Lett. 2013;15(14):3622-5. [PubMed] | [CrossRef] | [Google Scholar]
- Wacker DA, Kasireddy P. Efficient solid-phase synthesis of 2,3-substituted indoles. Tetrahedron Lett. 2002;43(29):5189-91. [CrossRef] | [Google Scholar]
- Bobko MA, Evans KA, Kaura AC, Shuster LE, Su DS. Synthesis of 2,5-disubstituted-3-cyanoindoles. Tetrahedron Lett. 2012;53(2):200-2. [CrossRef] | [Google Scholar]
- Sugino K, Yoshimura H, Nishikawa T, Isobe M. Regioselectivity of Larock indole synthesis using functionalized alkynes. Biosci Biotechnol Biochem. 2008;72(8):2092-102. [PubMed] | [CrossRef] | [Google Scholar]
- Gao Y, Shan D, Jia Y. Intramolecular Larock indole synthesis for the preparation of tricyclic indoles and its application in the synthesis of tetrahydropyrroloquinoline and Fargesine. ChemInform. 2014;70(34):5136-41. [CrossRef] | [Google Scholar]
- D’Souza DM, Müller TJ. Multi-component syntheses of heterocycles by transition-metal catalysis. Chem Soc Rev. 2007;36(7):1095-108. [PubMed] | [CrossRef] | [Google Scholar]
- Egger J, Carreira EM. Efficient synthesis strategies by application of transition metal-catalyzed carbene/nitrene insertions into C-H bonds. Nat Prod Rep. 2014;31(4):449-55. [PubMed] | [CrossRef] | [Google Scholar]
- Luck K, Modler KH. Synthesis of guidance mechanisms. Mech Mach Theor. 1994;29(4):525-33. [CrossRef] | [Google Scholar]
- Cabrera JA, Simón A, Prado M. Optimal synthesis of mechanisms with genetic algorithms. Mech Mach Theor. 2002;37(10):1165-77. [CrossRef] | [Google Scholar]
- Park Y, Kim Y, Chang S. Transition metal-catalyzed C-H amination: scope, mechanism and applications. Chem Rev. 2017;117(13):9247-301. [PubMed] | [CrossRef] | [Google Scholar]
- Lund H, Daasbjerg K, Lund T, Pedersen SU. On electron transfer in aliphatic nucleophilic substitution. Acc Chem Res. 1995;28(7):313-9. [CrossRef] | [Google Scholar]
- Mąkosza M. Electrophilic and nucleophilic aromatic substitutions are mechanistically similar with opposite polarity. Chemistry. 2020;26(67):15346-53. [PubMed] | [CrossRef] | [Google Scholar]
- Mąkosza M, Wojciechowski K. Application of vicarious nucleophilic substitution in organic synthesis. Liebigs Ann. 1997;1997(9):1805-16. [CrossRef] | [Google Scholar]
- Kolodiazhnyi OI. Stereochemistry of electrophilic and nucleophilic substitutions at phosphorus. Pure Appl Chem. 2019;91(1):43-57. [CrossRef] | [Google Scholar]
- Andrés J, Berski S, Domingo LR, González-Navarrete P. Nature of the ring-closure process along the rearrangement of octa-1,3,5,7-tetraene to cycloocta-1,3,5-triene from the perspective of the electron localization function and catastrophe theory. J Comp Chem. 2012;33(7):748-56. [PubMed] | [CrossRef] | [Google Scholar]
- Holub N, Blechert S. Ring-rearrangement metathesis. Chem Asian J. 2007;2(9):1064-82. [PubMed] | [CrossRef] | [Google Scholar]
- Jana S, Clements MD, Sharp BK, Zheng N. Fe(II)-catalyzed amination of aromatic C-H bonds via ring opening of 2H-azirines: synthesis of 2,3-disubstituted indoles. Org Lett. 2010;12(17):3736-9. [PubMed] | [CrossRef] | [Google Scholar]
- Maehara A, Tsurugi H, Satoh T, Miura M. Regioselective C-H functionalization directed by a removable carboxyl group: palladium-catalyzed vinylation at the unusual position of indole and related heteroaromatic rings. Org Lett. 2008;10(6):1159-62. [PubMed] | [CrossRef] | [Google Scholar]
- Lv J, Wang B, Yuan K, Wang Y, Jia Y. Regioselective direct C-4 functionalization of indole: total syntheses of (-)-agroclavine and (-)-elymoclavine. Org Lett. 2017;19(13):3664-7. [PubMed] | [CrossRef] | [Google Scholar]
- Zhang ZZ, Liu B, Wang CY, Shi BF. Cobalt(III)-catalyzed C2-selective C-H Alkynylation of indoles. Org Lett. 2015;17(16):4094-7. [PubMed] | [CrossRef] | [Google Scholar]
- Lanke V, Bettadapur KR, Prabhu KR. Site-selective addition of maleimide to indole at the C-2 position: Ru(II)-catalyzed C-H activation. Org Lett. 2015;17(19):4662-5. [PubMed] | [CrossRef] | [Google Scholar]
- Cacchi S, Fabrizi G. Synthesis and functionalization of indoles through palladium-catalyzed reactions. Chem Rev. 2005;105(7):2873-920. [PubMed] | [CrossRef] | [Google Scholar]
- Ghosh P, Das S. The C-H functionalization of N-alkoxycarbamoyl indoles by transition metal catalysis. Org Biomol Chem. 2021;19(37):7949-69. [PubMed] | [CrossRef] | [Google Scholar]
- Moriyama K, Ishida K, Togo H. Regioselective C(sp2)-H dual functionalization of indoles using hypervalent iodine(III): bromo-amination via 1,3-migration of imides on indolyl(phenyl)iodonium imides. Chem Commun (Camb). 2015;51(12):2273-6. [PubMed] | [CrossRef] | [Google Scholar]
- Yu W, Du Y, Zhao K. PIDA-mediated oxidative C-C bond formation: novel synthesis of indoles from N-aryl enamines. Org Lett. 2009;11(11):2417-20. [PubMed] | [CrossRef] | [Google Scholar]
- Vargas DA, Tinoco A, Tyagi V, Fasan R. Myoglobin-catalyzed C-H functionalization of unprotected indoles. Angew Chem Int Ed Engl. 2018;57(31):9911-5. [PubMed] | [CrossRef] | [Google Scholar]
- Poteat CM, Lindsay VN. Controlled α-mono- and α,α-di-halogenation of alkyl sulfones using reagent-solvent halogen bonding. Chem Commun (Camb). 2019;55(20):2912-5. [PubMed] | [CrossRef] | [Google Scholar]
- Guilbaud J, Selmi A, Kammoun M, Contal S, Montalbetti C, Pirio N, et al. C-H halogenation of pyridyl sulfides avoiding the sulfur oxidation: A direct catalytic access to sulfanyl polyhalides and polyaromatics. ACS Omega. 2019;4(24):20459-69. [PubMed] | [CrossRef] | [Google Scholar]
- Cerfontain H, Koeberg-Telder A, Laali K, Lambrechts HJ, Wit PD. Aromatic sulfonation. Halogen directing and steric effects in the sulfonation of the twelve halogenotoluenes and some related compounds. Recl Trav Chim Pays-Bas. 2010;101:390-2. [CrossRef] | [Google Scholar]
- Suter C, Weston AW. Direct sulfonation of aromatic hydrocarbons and their halogen derivatives. Org React. 2011:141-97. [CrossRef] | [Google Scholar]
- Heravi M, Rohani S, Zadsirjan V, Zahedi N. Fischer indole synthesis applied to the total synthesis of natural products. RSC Adv. 2017;7(83):52852-87. [CrossRef] | [Google Scholar]
- Cacchi S, Fabrizi G. Synthesis and functionalization of indoles through palladium-catalyzed reactions. Chem Rev. 2005;105(7):2873-920. [PubMed] | [CrossRef] | [Google Scholar]
- Mondal D, Kalar PL, Kori S, Gayen S, Das K. Recent developments on synthesis of indole derivatives through green approaches and their pharmaceutical applications. Curr Org Chem. 2020;24(22):2665-93. [CrossRef] | [Google Scholar]
- Mohammadi Ziarani GM, Moradi R, Ahmadi T, Lashgari N. Recent advances in the application of indoles in multicomponent reactions. RSC Adv. 2018;8(22):12069-103. [PubMed] | [CrossRef] | [Google Scholar]
- Kumar S, Ritika. A brief review of the biological potential of indole derivatives. Future J Pharm Sci. 2020;6(1):121 [CrossRef] | [Google Scholar]
- Saini T, Kumar S, Narasimhan B. Central nervous system activities of indole derivatives: an overview. Cent Nerv Syst Agents Med Chem. 2015;16(1):19-28. [PubMed] | [CrossRef] | [Google Scholar]
- Kumar D, Sharma S, Kalra S, Singh G, Monga V, Kumar B, et al. Medicinal perspective of indole derivatives: recent developments and structure-activity relationship studies. Curr Drug Targets. 2020;21(9):864-91. [PubMed] | [CrossRef] | [Google Scholar]
- Bugaenko DI, Karchava AV, Yurovskaya MA. Synthesis of indoles: recent advances. Russ Chem Rev. 2019;88(2):99-159. [CrossRef] | [Google Scholar]
- Colella M, Degennaro L, Luisi R. Continuous flow synthesis of heterocycles: A recent update on the flow synthesis of indoles. Molecules. 2020;25(14):3242 [PubMed] | [CrossRef] | [Google Scholar]
- Mancuso R, Dalpozzo R. Recent progress in the transition metal catalyzed synthesis of indoles. Catalysts. 2018;8(10):458 [CrossRef] | [Google Scholar]
- Barluenga J, Rodríguez F, Fañanás FJ. Recent advances in indole syntheses: new routes for a classic target. Chem Asian J. 2009;4(7):1036-48. [PubMed] | [CrossRef] | [Google Scholar]