ABSTRACT
Titanium dioxide nanoparticles, or TiO2 NPs, have shown potential in the fight against a range of diseases, including bacteria, viruses, fungi and parasites. Doping metals with nanoparticles modify their size and magnetic characteristics by decreasing the energy band gap and mixing spins and electrical charges. Furthermore, it has been shown that owing to their higher surface-to-volume ratio and improved ability to interact with bacterial cells, smaller metal-doped nanoparticles have better antibacterial action. Due in large part to their production, TiO3-NPs have outstanding antifungal and antibacterial capabilities. The major mechanism of TiO3-NPs’ antibacterial activity is their potent oxidizing capacity, which produces superoxide and hydroxyl anion radicals. Pseudomonas aeruginosa, Staphylococcus aureus and Escherichia coli are just a few of the pathogens against which TiO2 NPs have been shown to have antibacterial ability. Review topics will include the most recent discoveries in the sectors of water treatment, agriculture and medicine as well as the synthesis of TiO3- nanoparticles and their antimicrobial actions. TiO2 NPs as antibacterial agents: prospects and challenges will also be highlighted in the review.
INTRODUCTION
Antimicrobial Resistance (AMR) undermines the effectiveness of currently available antibiotics; it poses a serious threat to both human health and the global health community. It is critically important to develop new tactics and effective antimicrobial agents in this constantly changing fight against harmful bacteria. One of the most promising alternatives is titanium dioxide nanoparticles (TiO2 NPs) doped with metal; their exceptional antibacterial activity against a variety of microorganisms has drawn attention to them. This thorough analysis explores the intriguing world of these nanoparticles, elucidating their modes of action, demonstrating their effectiveness against a range of infections and critically evaluating their potential in the fight against AMR.1 Because of its photocatalytic activity, pure titanium dioxide has been shown to have innate antibacterial qualities. When Ultraviolet (UV) light interacts with TiO2 Nanoparticles (NPs), it produces Reactive Oxygen Species (ROS) such as Hydroxyl radicals (OH-) and superoxide anions (O2-), which are potent antibacterial agents that damage and eventually kill micro, organisms by damaging their membranes, DNA and cell walls. However, the main limitation of pristine TiO2 NPs is that it require UV light to activate, which limits their use in a lot of practical situations.2 The increasing incidence of microbial attacks in various industries, including food, pharmaceuticals, textiles, water purification and food packaging, prompts a continuous quest for novel antimicrobial compounds. Interest in the production of inorganic antimicrobial chemicals has developed due to the increased bacterial resistance to specific antibiotics and the toxicity of certain organic antimicrobials agents to human health. Because of their broad-spectrum antibacterial properties, metal as well as several combinations of metal oxides have garnered a lot of attention among these substances. However, because of their superior qualities resulting from a high surface area-to-volume ratio, nanoscale materials are broadly acceptable. Antimicrobial nanoparticles have demonstrated better and unique activities from their bulk properties.3 Additionally, the greater surface area that nanoparticles have in touch with bacteria amplifies their antibacterial properties.4
It has been demonstrated that TiO2 nanoparticles exhibit exceptional antifungal and antibacterial properties against different pathogens, like viruses, fungi, also Gram-positive and Gram-negative bacteria.5 Numerous investigations have demonstrated the increased antibacterial activity of TiO2 nanoparticles via metal doping, including co-doping with nitrogen and silver and the creation of unique hollow TiO2 nanospheres with antibacterial qualities.6 TiO2 may be categorized as amorphous or crystalline depending on its crystallinity.7 Three polymorphs of Titanium dioxide (TiO2) are known to be available: rutile, brookite and anatase. Because anatase has a higher photocatalytic activity than rutile and brookite, it is the one that is most frequently used for photocatalytic applications.8 While rutile has the smallest band gap (~3.0 eV), it often exhibits photocatalytic activity that is up to ten times lower than that of anatase. Even though pure brookite polymorphs may have more photocatalytic activity than the other two polymorphs, using them in heterogeneous photocatalysis is a difficult challenge because of their intricate production process. When compared to individual polymorphs, mixed phases of TiO2 showed considerably better photocatalytic activity.9
TiO2 has been extensively researched for its capacity to function as a photocatalyst, producing The light-induced inactivation of microorganisms caused by Reactive Oxygen Species (ROS).10 TiO2 is the most effective photocatalyst among the many types. It has been shown that numerous organic contaminants may be efficiently degraded by photocatalytic disinfection using TiO2. Because of its commercial availability and chemical stability, TiO2 has been utilized extensively in photocatalysis.11 Because of the randomly arranged particles, the amorphous form of titanium dioxide (TiO2), sometimes referred to as anatase, has an uneven shape and typically develops around 350°C. Between 500 and 800°C, anatase and brookite permanently change into the stable rutile polymorph. Compared to rutile and brookite, anatase exhibits the highest degree of photosensitivity of the three polymorphs.12–14
Definition and properties of Titanium Dioxide (TiO2)
Titanium dioxide (TiO2) Nanoparticles (NPs) have drawn a great deal of attention due to their unique characteristics and uses, especially in the biomedical industry. Although a lot of research has been done on chemically manufactured TiO2 NPs, microbial production has a number of benefits over it, such as lower toxicity, biocompatibility and environmental friendliness. By biotransformation titanium precursors and using their metabolites, biomolecules such as enzymes as stabilizing and capping agents, microorganisms including bacteria, fungus and algae create TiO2 NPs.15 One major drawback is the effectiveness and efficiency of these microbially generated TiO2 NPs as a nano-photocatalyst or antibacterial agent.16 This is because different microbial proteins that are capped on the microbially generated TiO2 NPs (which have previously been shown in the literature) prevent the TiO2 NPs from doing their intended function. There is less contact between pathogens and TiO2 NPs or between pollutants and TiO2 NPs as a consequence of the biomolecule masking the active sites of TiO2 NPs during their antibacterial activity and photocatalytic impact. Furthermore, bigger biological macromolecules-up to several kilodaltons in size-are capped on the microbially produced TiO2 NPs, increasing the total size of the particles. Because biological macromolecules are larger than TiO2 NPs, the pathogens cannot be destroyed photocatalytically by them, which significantly reduces their entry into the pathogens. These TiO2 NPs produced by microbiology are not as effective as TiO2 NPs produced chemically or physically as antimicrobial agents or nano-photocatalysts.16
Depending on the synthesis technique used, Titanium dioxide (TiO2) Nanoparticles (NPs) may have different sizes. TiO2 NPs produced by microbial synthesis may have diameters ranging from 10-30 nm to over 100 nm, while NPs produced by chemical synthesis can have sizes between 10 and 100 nm.15 TiO2 nanoparticles may be produced using different kind of methods, including the sol-gel method,, solvothermal method, immediate synthesis method, simple mixing method, method, microwave-assisted synthesis and precipitation method, according to the results from the cited publication.17 In general, the synthesis technique may affect the size of TiO2 NPs, but other elements including surface chemistry and crystalline structure are also very important in defining their characteristics and uses.
Experimental techniques for metal-doped TiO2, SOL-GEL TiO2 by using different metal nanoparticles
It is seen that metal-doped titanium dioxide nanoparticles increase antibacterial effectiveness.18–20 TiO2’s photocatalytic activity is increased by doping it with different elements because new energy levels arise near to the conduction band. The heterogeneous process of photocatalysis using titanium dioxide depends critically on the catalyst’s surface.21 During the synthesis process, metal ions are added to the TiO2 lattice to create metal-doped Titanium dioxide (TiO2) Nanoparticles (NPs). Zn and Cd are examples of non-transition metals, while transition metals like Fe, Cu and Ni may be the dopants.22 TiO2 NPs may be doped to increase their photocatalytic activity, stability and adjustable bandgap characteristics, which will increase their efficiency in breaking down pollutants and producing hydrogen.9 There are numerous approaches to synthesize metal-doped TiO2 NPs, also solvothermal, sol-gel and hydrothermal approaches. The environmentally benign and biocompatible method of microbial synthesis has also been used to create metal-doped TiO2 NPs.23 However, TiO2’s antibacterial activity may be improved by doping it with metals like Silver (Ag), Nickel (Ni), Copper (Cu), Manganese (Mn) and Sulfur (S), particularly when exposed to visible light. Under visible light, Ag-doped TiO2 nanoparticles exhibited increased antibacterial activity against Staphylococcus aureus. TiO2 NPs doped with Ni, Cu, Mn and S have also demonstrated antibacterial activity against various bacterial species. Table 1 show TiO2-materials doped with metals has antibacterial properties against a variety of microorganisms. Moreover, it has been discovered that adding metal-doped TiO2 nanoparticles to elastomers improves the photoinactivation effectiveness of visible light for surface cleaning. These results imply that metal-doped TiO2 nanoparticles may find usage in a variety of applications as powerful antibacterial agents.
Metal Dopant | Bacteria Tested | Inhibition Zone (mm) or MIC | References |
---|---|---|---|
Ag | Staphylococcus. aureus, Escherichia coli. | 10-20 mm; MIC=0.05 mg/mL. | 24 |
Cu | Bacillus subtilis, Pseudomonas aeruginosa. | 15-20 mm; MIC=0.1-0.5 mg/mL. | 25 |
Zn | Staphylocccus aureus, Klebsiella pneumoniae, Pseudomonas aeruginosa. | 10-20 mm; MIC=0.1-0.5 mg/mL. | 26 |
Cr | Staphylococcus aureus, Escherichia coli, Pseudomonas aeruginosa. | 10.5-19.25 mm; MIC=31.25 ug/mL. | 27 |
TiO2-materials doped with metals have antibacterial properties against a variety of microorganisms.
Experimental techniques for metal-doped TiO2, sol-gel TiO2/Ni synthesis and antibacterial testing
Sol-gel TiO2/Ni synthesis and antibacterial testing
According to Haque et al. (2023) the compound’s synthesis has concluded. For the procedure TiO2 nanoparticles were produced via sol-gel using titanium isopropoxide as the titania precursor. A homogeneous 1:1 (v/v) mixture of water and 2-proponal was combined with a solution of titanium isopropoxide (0.1 M) in 2-proponal (25 mL), added drop by drop and constantly stirred. Turbidity in the solution would emerge as a consequence, indicating the hydrolysis of titanium isopropoxide. Following the full addition, the mixture was shaken for the whole night before being filtered and repeatedly cleaned with water. In order to dope TiO2 particles with nickel, a solution including water and 2-proponal was mixed with a known concentration of NiCl2 (0.1-0.8%) before being added to the titanium isopropoxide solution. First, the undoped TiO2 and Ni-TiO2 were both dried for 2-3 hr at 100 C. After the dry residue was manually ground in an agate mortar, it was heated to various temperatures-300, 400 and 500°C-for 4 hr. Figure 1 demonstrate the sol-gel method’s synthesis of metal-doped TiO2/Ag.28 Figure 1 show the Synthesis of metal-doped TiO2/Ni by sol-gel method.
Characterization of TiO2/Ni
Using a range of analytical methods, the structural, morphological and elemental properties of TiO2/Ni nanocomposites prepared by the sol-gel process are examined. Numerous techniques, including as X-ray Diffraction (XRD), Scanning Electron Microscopy (SEM), energy dispersive X-ray Spectroscopy (EDX), Fourier Transform Infrared Spectroscopy (FTIR), etc., are often used to study TiO2/Ni nanocomposites.29 SEM, XRD and UV-vis spectroscopy were among the traditional analytical techniques used to characterize the generated catalysts. To study the particle morphology, a Hitachi S-5000 FEG scanning electron microscope running at 20 kV was used. Using Cu Kα radiation (h=1.5418 ù) from a Rigaku Miniflex II device running at 30 kV and 15 mA, X-ray Diffraction (XRD) was used to structurally characterize the undoped TiO2 and Ni-TiO2 nanoparticles in the 20-80° range. Using a Shimadzu UV-vis spectrophotometer (Model 1601), the UV-Vis spectra in the 300-800 nm range was obtained at room temperature 28
Antibacterial Effects
Staphylococcus aureus is strongly inhibited by Ni-doped TiO2 nanoparticles. The antibacterial activity was measured using the well diffusion method. After being prepared, cleaned and put into a petri plate, Mueller-Hinton agar (Hi-Media) was used. After allowing the medium to solidify, the aseptic placement of the prepared inoculum onto the plate occurred. Using a cotton swab or an L-shaped glass spreader that had been sterilized, the inoculum was applied. A sterilized test tube was used to create a well with a 6 mm diameter, which was then filled with a 2.5 L suspension of various diluted products to be tested. At 28 degrees, the plates were incubated for three days. The zone of inhibition diameter (mm) is used to represent the activity. Figure 2 shows how different concentrations of the Ni dopant TiO2 affected antibacterial activity. With an increase in dopant concentration, the zone of inhibition widened. However, it was shown that agglomeration caused the zone of inhibition to narrow at a concentration of 2%.30
Experimental techniques for metal-doped TiO2, sol-gel TiO2/Ag synthesis and antibacterial testing
Nanoparticles composed of Ag-TiO2 were synthesized through a range of diverse methodologies as described in the investigations conducted by Fan et al.,31 de Oliveira et al.,18 Wani et al. and Ren et al.32 Fan and colleagues conducted a synthesis of a composite material that consisted of Polyamino Acid (PAA) and TiO2/Ag nanoparticles via in situ melting polycondensation.33 Ag-doped TiO2 nanoparticles were synthesized by de Oliveira et al. using the sol-gel process followed by Ag doping. Wani et al. looked into the use of ion beam irradiation to create N and Ag-modified nanocrystalline TiO2. Ren et al.’s attempt to create PPDO-coated Ag loading TiO2 nanoparticles was unsuccessful. These studies used a variety of approaches, including ion beam irradiation, sol-gel process and in situ melting polycondensation, to create Ag-TiO2 nanoparticles with different compositions and characteristics. Figure 3 show TiO2, sol-gel TiO2/Ag synthesis. The synthesis of Ag-TiO2 nanocomposites can be accomplished through a modified sol-gel technique, wherein the incorporation of silver ions takes place in the course of the preparation and undergoes reduction to generate Ag nanoparticles on the surface of TiO2.34,35
Using a nickel filter, powder X-ray Diffraction (XRD) spectra of anode Cu-WL 1 radiation with a wavelength of 1.5406 nm were acquired from PAN Analytical in order to assess the crystalline structure of the photocatalysts. The voltage was 40 kV and the current was 40 milliamperes. The average crystallite size of anatase was determined by using the Scherrer equation in combination with Full Width at Half Maximum (FWHM) data from the chosen peak. The surface area and porosity of the material were determined using the Micrometrics Gemini VII surface area analyzer. The nitrogen adsorption/desorption isotherms were monitored two or three times to get repeatable results. The data came from the surface/volume mesopore study of BJH. By using the Frenkel-Halsey-Hill isotherm equation, the micropores’ volume may be calculated. For duration of 2 hr, every sample was degassed at 300°C. A Transmission Electron Microscope (TEM) equipped with JEOL/JEM 2100 and running at 200 kV was used to measure the catalyst’s size. Using a Scanning Electron Microscope (SEM), the catalyst’s form and underlying elemental composition were examined.36 The researchers are Imandi Manga Raju, Siva Rao Tirukkovalluri and Sankara Rao Miditana.
The following reaction rate kinetic constants were determined using the pseudo-first-order kinetic model:
Where,
t is the irradiation time,
k is kinetic constant (min-1) and,
C is the concentration of the MB (mg/L).
Antibacterial action of amorphous and crystalline titanium doped with silver
In compliance with reference methods of the Clinical and Laboratory Standards Institute (CLSI) for the determination of MICs of aerobic bacteria by broth microdilution, the antibacterial activity of amorphous and crystalline TiO2:Ag0, TiO2a: Ag0, TiO2:Ag0/Ag+, TiO2:Ag0/Ag+ and TiO2:Ag0/ Ag0 nanospheres was evaluated using Minimal Inhibitory Concentration (MIC) and Minimal Bactericidal Concentration (MBC) values. The lowest concentration of silver on a microplate that prevented bacterial growth was known as the Minimum Inhibitory Concentration (MIC) and it was determined after 16-19 hr of incubation at 37°C. The lowest extract concentration that was found in MBC eliminated 99.9% of the germs.38 Two important microbiological indicators that indicate how well antimicrobial medications work against bacteria are the Minimum Bactericidal Concentration (MBC) and least Inhibitory Concentration (MIC) values. Lower MIC and MBC values, which indicate the lowest doses at which 99.9% of bacteria are killed and bacterial growth is inhibited, respectively, indicate better antibacterial efficacy. The Figure 4 show graphical statics of Antibacterial Action of Ni/TiO2 against Staphylococcus. Mueller Hinton Broth (MHB) and Mueller Hinton Agar (MHA) were utilized as the bacterial growth media throughout the experiment. 200 ml was dispensed each spot for testing and the antimicrobial agent stock solution was diluted by published methods. The Figure 4 show graphical statics of Antibacterial Action of Ni/TiO2 against Staphylococcus. A 0.5 McFarland standard containing around 1-2×10^8 Colony-Forming Units per milliliter (CFU/mL) was altered to generate the inoculum. To get the intended final inoculum concentration of 10^4 CFU per spot, this standard was then diluted at a ratio of 1:10 in sterile saline. Spots devoid of nanoparticles containing medium and cultures were included as controls to guarantee precise evaluation of the antimicrobial agent’s ability to impede bacterial growth and kill it.35
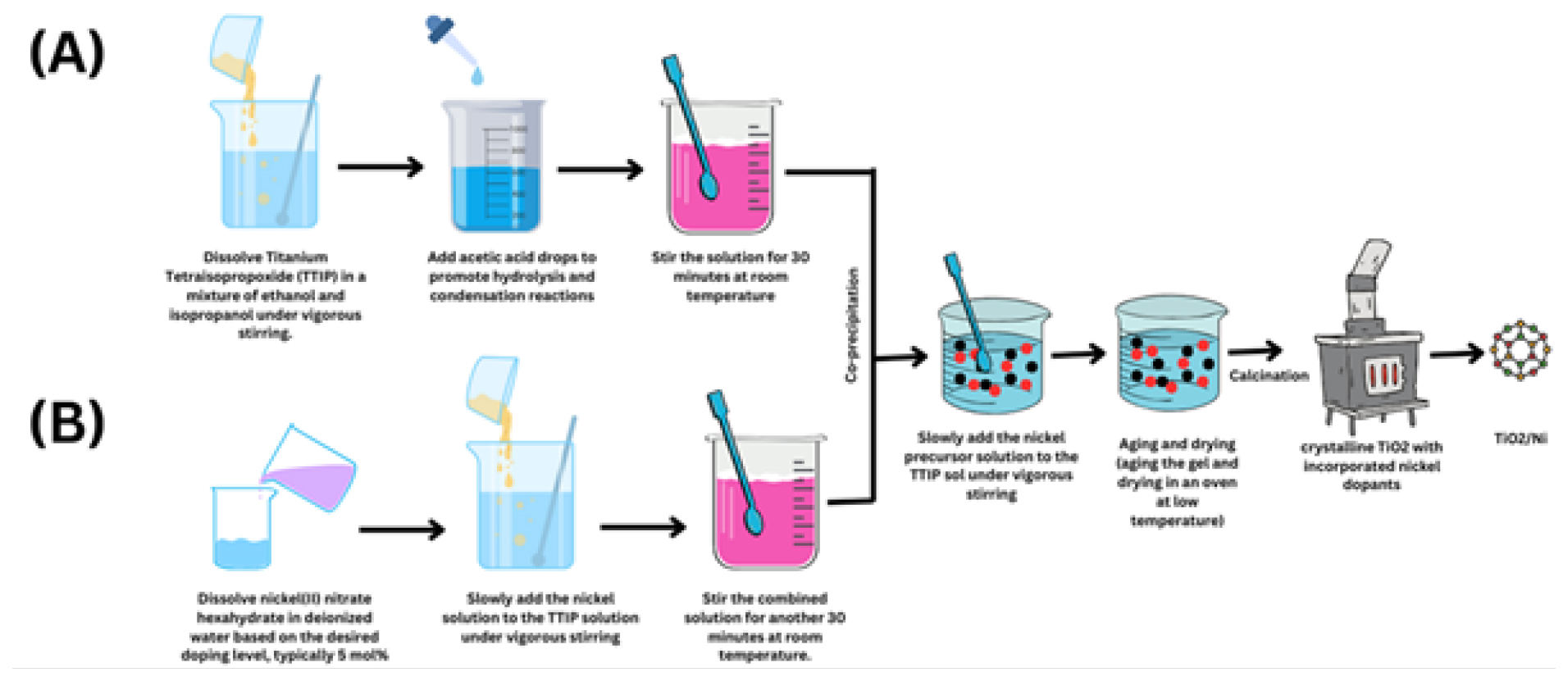
Figure 1:
Synthesis of metal-doped TiO2/Ni by sol-gel method.
Nanoparticles of doped titanium dioxide (TiO3-NPS)
TiO3-NPs, or nanoparticles of doped titanium dioxide, have drawn a lot of interest because of their special qualities and possible uses. The physicochemical characteristics of titanium dioxide nanoparticles vary from those of their fine particle analogs, potentially modifying their bioactivity Because of their stability and photocatalytic qualities, TiO2 NPs are extensively employed in many different sectors, including solar cells, water treatment and catalytic processes. Inhalation is the main way that people are exposed to TiO2 NPs at work, however, consumer goods like sunscreen and makeup may also expose people to these particles.39
TiO2 NPs may move from the lung and gastrointestinal tract to systemic organs, according to studies, which raises questions regarding possible health repercussions.40
Additionally, studies have concentrated on creating nitrogen-doped titanium dioxide nanoparticles, which exhibit greater absorbance in the visible spectrum than plain ones.41 There are many ways to synthesize titanium dioxide nanoparticles, one of which is green synthesis, which uses plant extracts and is safe for the environment and non-toxic. Applications in wastewater treatment and antibacterial activity have also been investigated for the microbial production of titanium dioxide nanoparticles.15
Various Uses of Doped-TiO3 in Biomedicine
Numerous applications for doped-TiO2 nanoparticles exist in biomedicine. They have been investigated as possible temperature nanoprobes, between the doped nanoparticles’ thermally sensitive radiative transitions, the fluorescence intensity ratio establishes the sensors’ relative sensitivity.42 Doped or undoped TiO2 nanoparticles have shown significant use in enhancing chemical, biological and physical behavior. They have been used to improve several facets of the environment, agriculture and health, such as enhancing nanomedicine, eliminating pollutants from the environment and enhancing agricultural qualities.43 Potential optical nanothermometers using Nd3+ doped TiO2 nanocrystals have been studied. Temperature is determined by the fluorescence intensity ratio of emissions at various wavelengths.44 The Figure 5 shows various applications of Various Uses of Doped-TiO3 in Biomedicine.
Additionally, doped-TiO2 nanoparticles have been used to produce novel biocompatible materials with distinct physical-chemical characteristics, such as coatings of magnetite nanoparticles with noble metals.45 Nitrogen-doped nanoparticles have demonstrated enhanced actions that include cytotoxic, antifungal, antioxidant, antidiabetic and inhibit protein kinase when compared to undoped TiO2 nanoparticles. As such, they represent a strong contender for biomedical applications.46
Current research on the use of doped-TiO3 in biomedical applications is centered on its utilization in ferroelectrics and luminous phosphors, among other functional materials. One well-known example is the development of a Mn- and Yb-doped Ba TiO3-(Na0.5Bi0.5) TiO3 ferroelectric relaxor material; the Mn doping results in better dielectric characteristics. This work suggests that Mn dopant functions as an acceptor, enhancing electrical resistivity in the vicinity of the Curie temperature and decreasing oxygen vacancies, which enhances insulation resistance.47
A further investigation delves into the creation and description of (Ba, Sr) TiO3 phosphors doped with Eu3+ and Dy3+, demonstrating that these dopants provide adjustable photoluminescence characteristics. The emission intensities of the phosphors change when Sr2+ is substituted for Ba2+, causing a phase shift from tetragonal to cubic structures.48
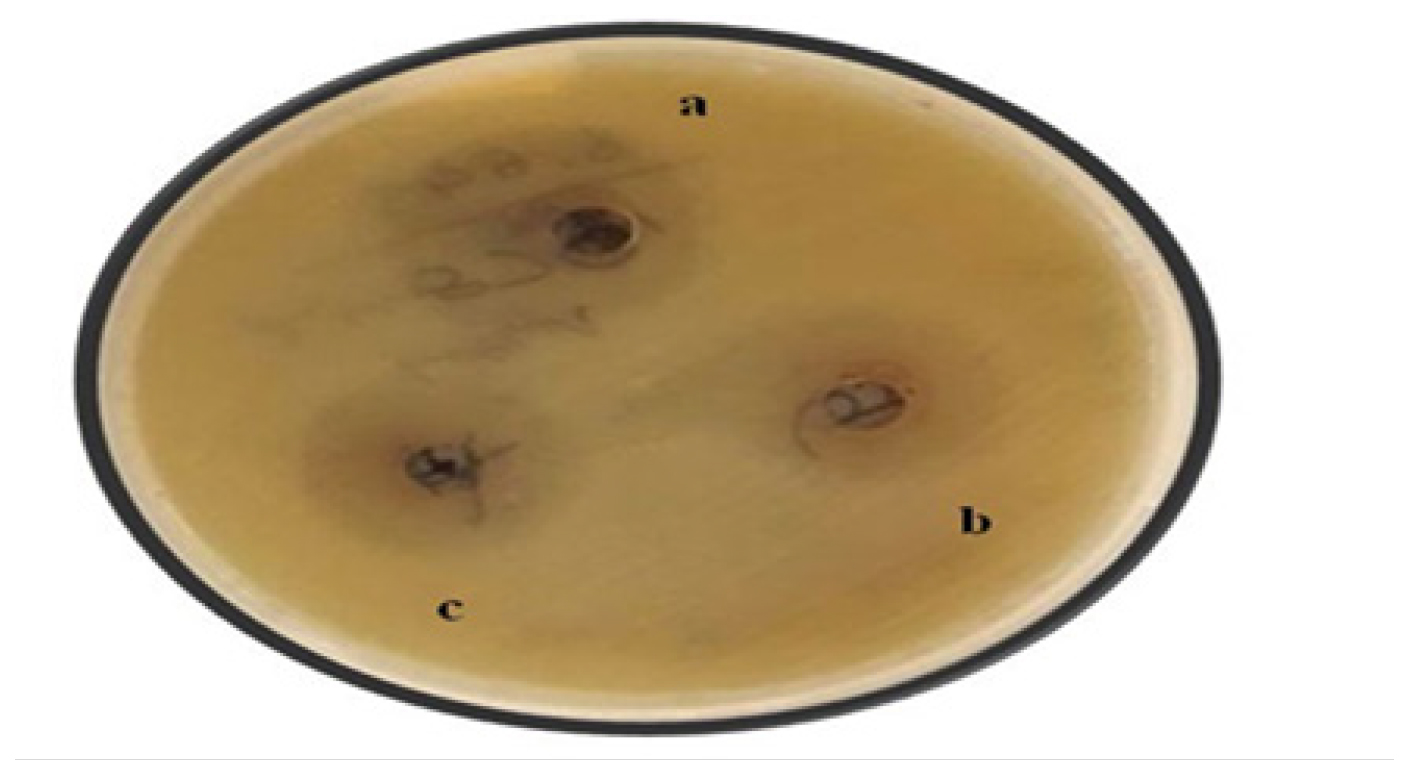
Figure 2:
Antibacterial effect of (a) 1% Ni/TiO2 (b) 1.5% Ni/TiO2 and (c) 2% Ni/TiO2 against Staphylococcus aureus. The figure is taken from.30
It is further shown that the structural, electrical and optical characteristics of the resultant materials may be studied by doping Y at the Ba and Ti sites of BaTiO2.49 Rare-earth element doping (Pr3+, Nd3+, Sm3+, Eu3+, Ho3+, Er3* and Yb3+) in lead-free ferroelectric Bi1/2Na1/2TiO3 materials has also been investigated to get adjustable photoluminescent characteristics.50
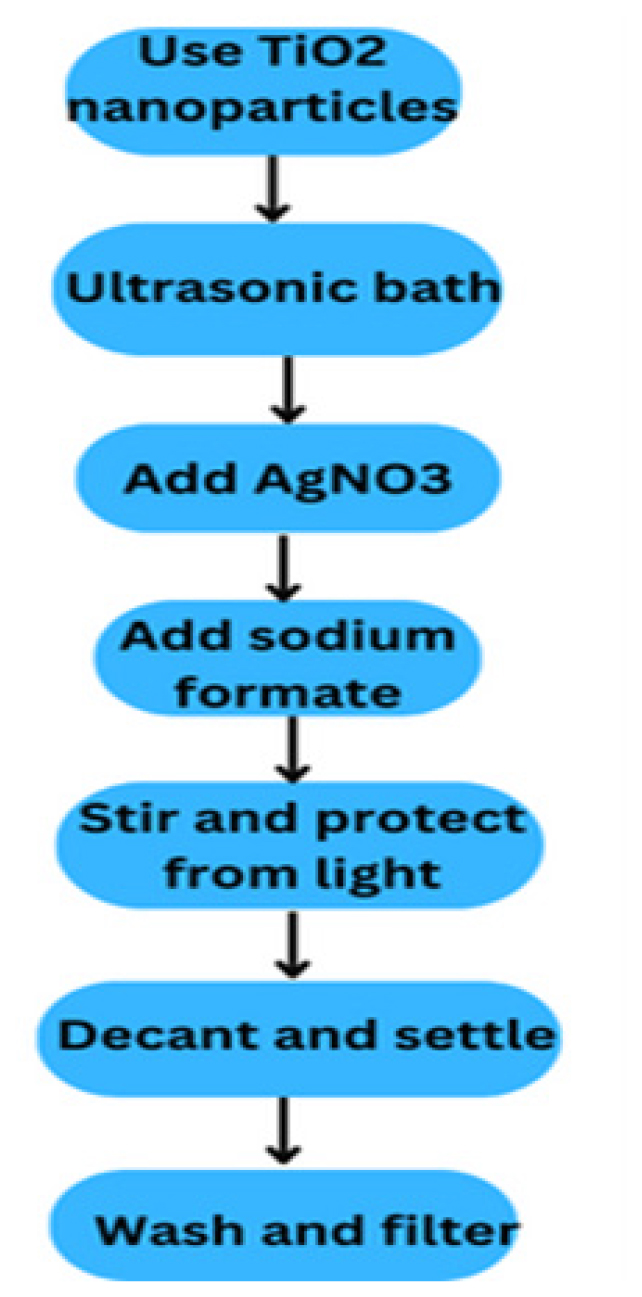
Figure 3:
TiO2, sol-gel TiO2/Ag synthesis.
Doped-TiO2-Based Antimicrobial Activities
Doped TiO2 nanoparticles have shown antimicrobial efficacy against a variety of bacteria under a range of lighting conditions. Under visible light, methicillin-resistant and susceptible Staphylococcus aureus was more effectively inhibited by Ag-doped TiO2 nanoparticles.18 Table 2 shows possible mechanisms and Doped Materials.
Doped Materials | Nanoparticles | Size (nm) | Synthesis Methods | Coating Materials | Organisms | Cone, of Nanoparticles (μg/mL) | ZOI (mm) | MIC (μg/mL) | MBC (μg/mL) | Mechanisms | References |
---|---|---|---|---|---|---|---|---|---|---|---|
Ag/TiO, | Silver/ Titanium Dioxide | 10-20 | Sol-gel | None | E. coli | 50 | 15 | 25 | 50 | Cell membrane disruption, ROS generation. | 54 |
Cu/TiO, | Copper/ Titanium Dioxide | 5-15 | Hydrothermal | Chitosan | S. aureus | 100 | 18 | 50 | 100 | DNA damage, protein denaturation. | 55 |
Zn/TiO, | Zinc/Titanium Dioxide | 20-30 | Coprecipitation | PEG | B. subtilis | 25 | 12 | 10 | 20 | Lipid peroxidation, oxidative stress. | 56 |
Fe/TiO, | Iron/Titanium Dioxide | 8-15 | Solvothermal | Silica | P. aeruginosa | 75 | 20 | 30 | 60 | Membrane damage, enzyme inhibition. | 57 |
Mechanism of actions of doped-TiO2 and possible mechanisms.
Escherichia coli and B. subtilis are two examples of the Gram-positive and Gram-negative bacteria that the ZnO-doped TiO2 nanocomposites shown increased antibacterial activity against.51 Furthermore, yttrium-doped TiO2 nanostructures showed exceptional antibacterial properties against E. coli.52 One can decrease electron-hole recombination, boost resistance to microorganisms and dope TiO2 with various ions or compounds to expand photocatalytic activity to visible light.29 Under visible light, it has been shown that metal-doped TiO2, e.g., TiO2/Ni, TiO2/Cu and TiO2/Mn/S, inhibits a variety of bacteria, including B. coagulans, K. pneumonia, E. coli and S. aureus.53
Doped-TIO2’S antimicrobial activity mechanisms
Doped-TiO2 has antibacterial action via a variety of methods. TiO2 may be doped with various ions or chemicals and compounded with polymers to increase its photocatalytic activity and range to visible light, hence improving its antibacterial effectiveness.18 ZnO-doped TiO2, one kind of etal-doped TiO2, has shown improved antibacterial activity against a range of microbes., such as Escherichia coli and Staphylococcus aureus.29 Figure 6 illustrates the Mechanism of actions of doped-TiO2. Doped-TiO2 has antibacterial properties because light exposure produces Reactive Oxygen Species (ROS), which may harm the cell membranes of bacteria and prevent bacterial development.51 To ensure the uniformity and control over mass distribution of doped TiO2 nanoparticles, the sol-gel production process is often used. TiO2 has strong antibacterial properties because of the synergistic effects of photocatalysis and the targeted activities of dopant compounds. Doped-TiO2 produces Reactive Oxygen Species (ROS) such as superoxide anions and hydroxyl radicals when exposed to light. These ROS led to oxidative stress in microbial cells, which harms proteins, lipids and DNA and finally causes cell death. Dopants such as zinc and copper also contribute extra antibacterial mechanisms. For example, copper ions may physically harm cell membranes and interfere with cellular metabolic processes. Doped-TiO2 is a very powerful substance that can effectively fight a variety of bacteria, viruses and fungi due to the combined effects of these methods.58
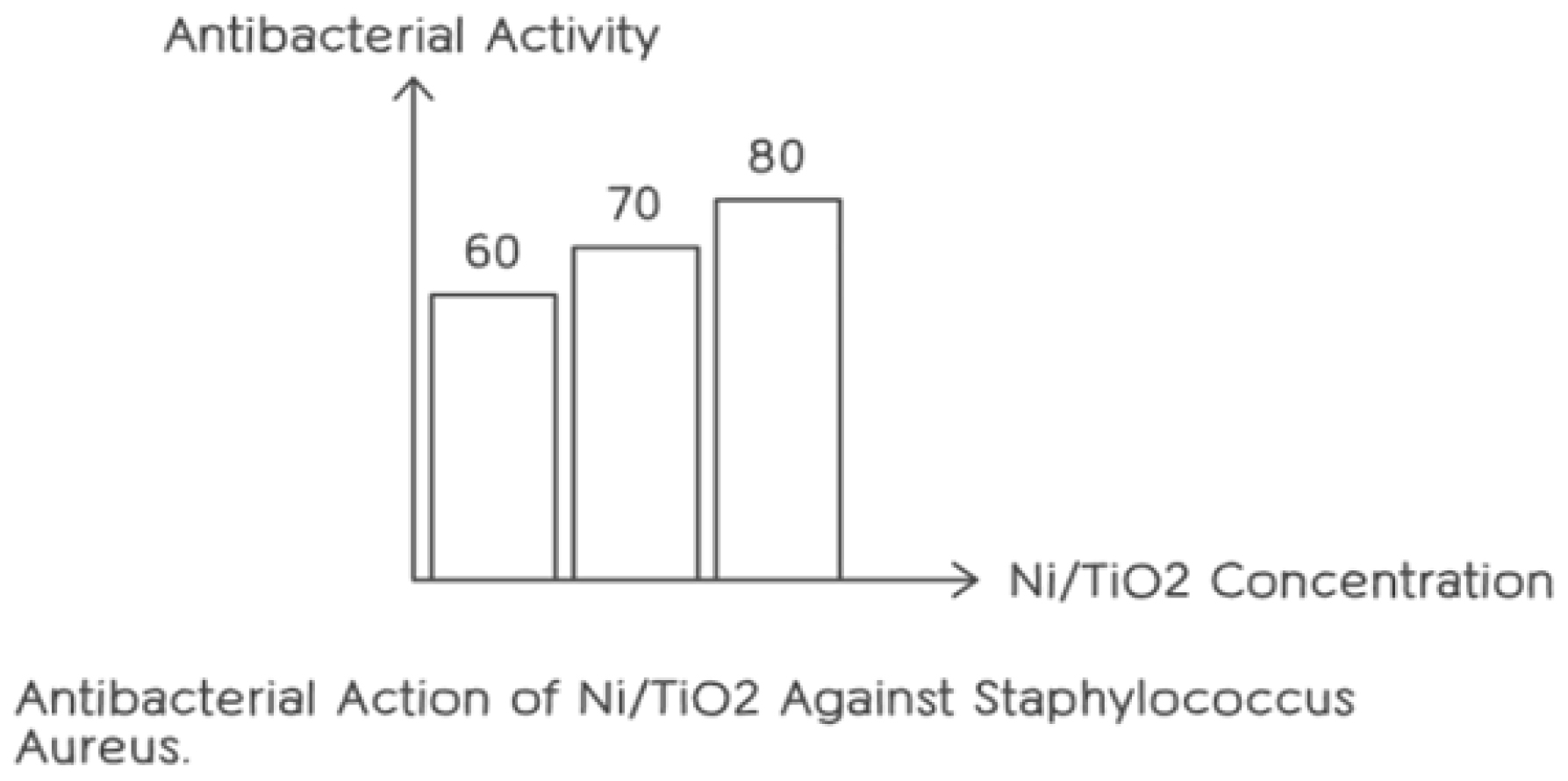
Figure 4:
Antibacterial Action of Ni/TiO2 against Staphylococcus.
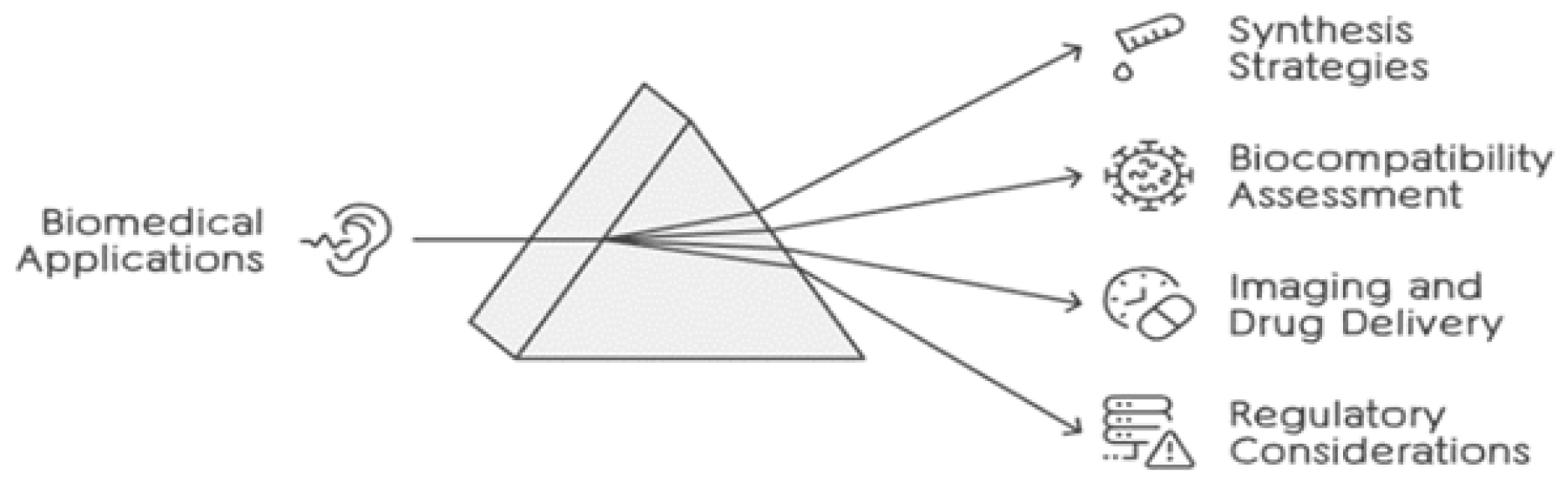
Figure 5:
Various Uses of Doped-TiO3 in Biomedicine.
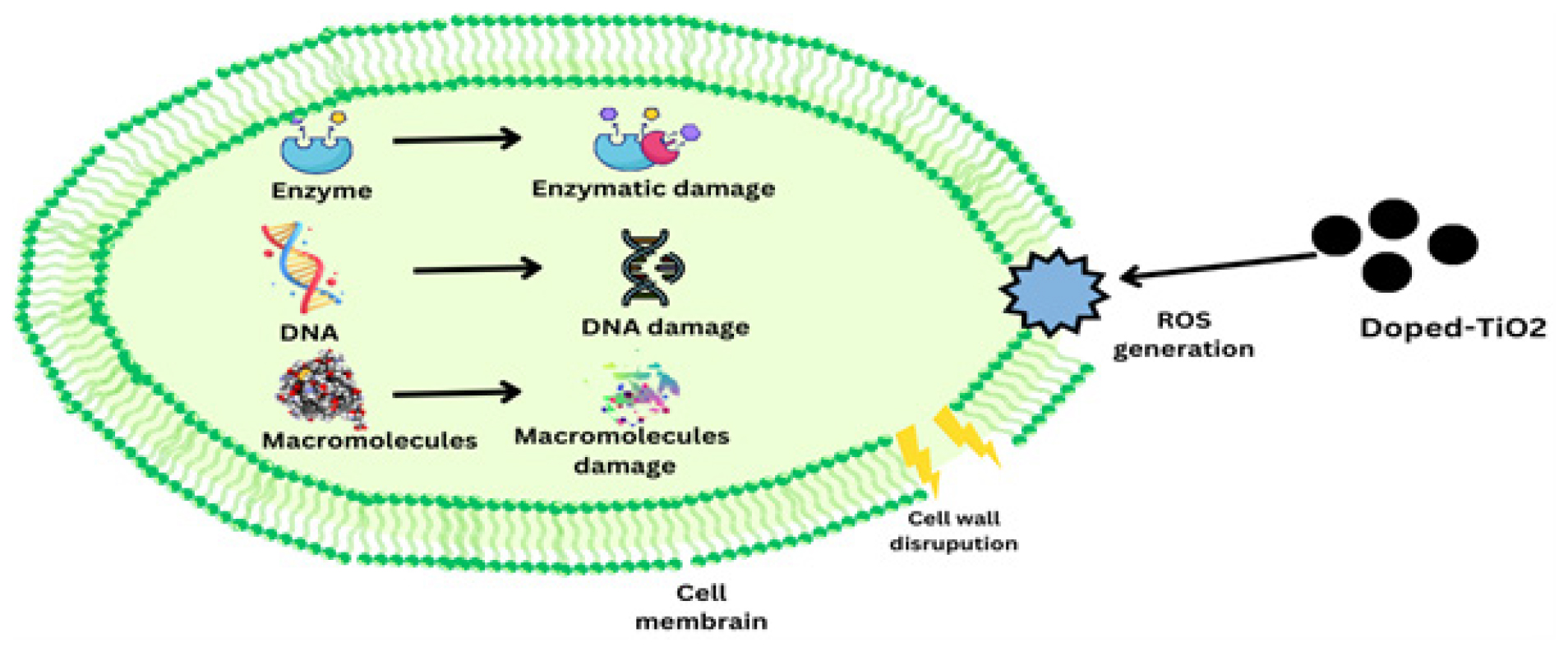
Figure 6:
Mechanism of actions of doped-TiO2
ROS generation
Numerous studies place a strong emphasis on the generation of Reactive Oxygen Species (ROS). A promising method for LDs-mediated photodynamic treatment was created by Wang et al. using a photosensitizer-based system that preferentially accumulates into Lipid Droplets (LDs) and produces ROS upon light activation.59 Palladium (Pd) nanocrystals with various crystalline forms were examined for their cytotoxicity by Wu et al. They discovered that facet-dependent ROS production, which is facilitated by Pd nanocrystals, is a critical component of cytotoxicity.60 A potential therapeutic approach has been presented by Ling et al.’s development of a biomimetic nanosheet material that can create ROS via a cascade of intracellular biological events and provide H2O2 in an acidic environment.61
Photoexcitation
Photon energy higher than or equal to the doped TiO2’s modified bandgap are absorbed by the substance. In the Valence Band (VB), a positive hole (h+) remains because an electron (e-) is promoted to the Conduction Band (CB).
The formula is as follows:
Dynamics of Charge Carriers
Dopants add trap sites that catch holes or electrons, preventing their recombination and boosting the effectiveness of following processes that generate ROS.63
ROS Formation
Holes react when they come into contact with hydroxide ions or adsorbed water molecules.
Electron reactions: Electrons cut down on molecules of dissolved oxygen.
CONCLUSION
Metal-doped titanium dioxide nanoparticles have demonstrated amazing potential as an antibacterial agent against a range of illnesses. Doping metals on top of nanoparticles can change their size and magnetic characteristics by mixing spins and electrical charges to reduce the energy band gap. Additionally, it has been demonstrated that because of their larger surface-to-volume ratio and enhanced ability to interact with bacterial cells, smaller metal-doped nanoparticles exhibit stronger antibacterial action. TiO2 NPs have remarkable antifungal and antibacterial properties. Their primary mode of action against bacteria is attributed to their strong oxidizing ability, which generates radicals such as superoxide and hydroxyl anion. TiO2 NPs doped with metals, namely silver, nickel, copper, manganese and sulfur, have shown increased antibacterial activity against several kinds of bacteria. Sol-gel, solvothermal and hydrothermal techniques are some of the ways that may be used to synthesize metal-doped TiO2 NPs. It is possible to assess the antibacterial activity of metal-doped TiO2 NPs utilizing methods like the well diffusion technique. All things considered, metal-doped titanium dioxide nanoparticles have the potential to be an effective antibacterial agent in a variety of fields, such as medicine, agriculture and water treatment.
Cite this article:
Sheikh M, Makkad S, Shende S, Deshmukh M. Antimicrobial Efficacy of Metal-Doped Titanium Dioxide Nanoparticles: A Comprehensive Review. Int. J. Pharm. Investigation. 2024;14(4):1042-51.
ACKNOWLEDGEMENT
I would like to express my gratitude to the Co-authors specifically Saniya Makkad, Sanskruti Shende, and Mrunal Deshmukh, for their comprehensive review on the antimicrobial efficacy of metal-doped titanium dioxide nanoparticles.
ABBREVIATIONS
UV | Ultraviolet ROS: Reactive Oxygen Species |
---|---|
OH | Hydroxyl radicals |
O2 | Superoxide anions |
MIC | Minimal Inhibitory Concentration |
MBC | Minimal Bactericidal Concentration |
CFU/mL | Colony-Forming Units per milliliter |
CLSI | Clinical and Laboratory Standards Institute |
MHB | Mueller Hinton Broth |
MHA | Mueller Hinton Agar |
TEM | Transmission Electron Microscope |
SEM | Scanning Electron Microscope |
BJH | Brunauer, Emmett, and Teller |
FHH | Frenkel-Halsey-Hill |
References
- Hajipour MJ, Fromm KM, Akbar Ashkarran A. Antibacterial properties of nanoparticles. Trends Biotechnol. 2012;30(10):499-511. [CrossRef] | [Google Scholar]
- Younis AB, Haddad Y, Kosaristanova L, Smerkova K. Titanium dioxide nanoparticles: Recent progress in antimicrobial applications. WIREs Nanomedicine Nanobiotechnology. 2023;15(3) [CrossRef] | [Google Scholar]
- Whitesides GM. Nanoscience, Nanotechnology and Chemistry. Small. 2005;1(2):172-9. [CrossRef] | [Google Scholar]
- Chen MC, Koh PW, Ponnusamy VK, Lee SL. Titanium dioxide and other nanomaterials based antimicrobial additives in functional paints and coatings: Review. Prog Org Coat. 2022;163:106660 [CrossRef] | [Google Scholar]
- . Antimicrobial Resistance-A One Health Perspective. 2021 [CrossRef] | [Google Scholar]
- Anbumani D, Dhandapani KV, Manoharan J. Green synthesis and antimicrobial efficacy of titanium dioxide nanoparticles using leaf extract. J King Saud Univ – Sci. 2022;34(3):101896 [CrossRef] | [Google Scholar]
- Chakravarty P, Deka H, Chowdhury D. Anthracene removal potential of green synthesized titanium dioxide nanoparticles (TiO2-NPs) and Alcaligenes faecalis HP8 from contaminated soil. Chemosphere. 2023;321:138102 [CrossRef] | [Google Scholar]
- Nam Y, Lim JH, Ko KC, Lee JY. Photocatalytic activity of TiO nanoparticles: a theoretical aspect. J Mater Chem A. 2019;7(23):13833-59. [CrossRef] | [Google Scholar]
- Anucha CB, Altin I, Bacaksiz E, Stathopoulos VN. Titanium dioxide (TiO2)-based photocatalyst materials activity enhancement for Contaminants of Emerging Concern (CECs) degradation: In the light of modification strategies. Chem Eng J Adv. 2022;10:100262 [CrossRef] | [Google Scholar]
- Foster HA, Ditta IB, Varghese S, Steele A. Photocatalytic disinfection using titanium dioxide: spectrum and mechanism of antimicrobial activity. Appl Microbiol Biotechnol. 2011;90(6):1847-68. [CrossRef] | [Google Scholar]
- Jain A, Vaya D. PHOTOCATALYTIC ACTIVITY OF TiO2 NANOMATERIAL. J Chil Chem Soc. 2017;62(4):3683-90. [CrossRef] | [Google Scholar]
- Žerjav G, Žižek K, Zavašnik J, Pintar A. Brookite vs. rutile vs. anatase: What’s behind their various photocatalytic activities?. J Environ Chem Eng. 2022;10(3):107722 [CrossRef] | [Google Scholar]
- Luttrell T, Halpegamage S, Tao J, Kramer A, Sutter E, Batzill M, et al. Why is anatase a better photocatalyst than rutile? – Model studies on epitaxial TiO2 films. Sci Rep. 2014;4(1):4043 [CrossRef] | [Google Scholar]
- Zhang J, Zhou P, Liu J, Yu J. New understanding of the difference of photocatalytic activity among anatase, rutile and brookite TiO2. Phys Chem Chem Phys. 2014;16(38):20382-6. [CrossRef] | [Google Scholar]
- Rathore C, Yadav VK, Gacem A. Microbial synthesis of titanium dioxide nanoparticles and their importance in wastewater treatment and antimicrobial activities: a review. Front Microbiol. 2023;14:1270245 [CrossRef] | [Google Scholar]
- Yang R, Hou E, Cheng W. Membrane-Targeting Neolignan-Antimicrobial Peptide Mimic Conjugates to Combat Methicillin-Resistant (MRSA) Infections. J Med Chem. 2022;65(24):16879-92. [CrossRef] | [Google Scholar]
- Seman N, Tarmizi ZI, Ali RR. Preparation Method of Titanium Dioxide Nanoparticles and Its Application: An Update. IOP Conf Ser Earth Environ Sci. 2022;1091(1):012064 [CrossRef] | [Google Scholar]
- Oliveira IND, Oliveira RIS, Pereira EB, Padilha FF, Egues SM, Hernández-Macedo ML, et al. Synthesis and photocatalytic effects of TiO2-Ag on antibiotic-resistant bacteria. Peer Rev. 2023;5(8):45-61. [CrossRef] | [Google Scholar]
- Hazem Najem A, Mahmood Khudhur I, M A, Ali G. Inhibitory effect of Titanium dioxide (TiO2) nanoparticles and their synergistic activity with antibiotics in some types of bacteria. Bionatura. 2023;8(1):1-7. [CrossRef] | [Google Scholar]
- McShea L, Kambo HS, Maclean M, Sandison ME. Metal oxide-doped elastomeric materials for amplifying visible light-based antimicrobial activity. Mater Res Express. 2022;9(8):85402 [CrossRef] | [Google Scholar]
- Khlyustova A, Sirotkin N, Kusova T, Kraev A, Titov V, Agafonov A, et al. Doped TiO2: the effect of doping elements on photocatalytic activity. Mater Adv. 2020;1(5):1193-201. [CrossRef] | [Google Scholar]
- Prakash J, Samriti Kumar A. Novel rare earth metal-doped one-dimensional TiO2 nanostructures: Fundamentals and multifunctional applications. Mater Today Sustain. 2021;13:100066 [CrossRef] | [Google Scholar]
- Oganisian K, Hreniak A, Sikora A, Gaworska-Koniarek D, Iwan A. Synthesis of iron doped titanium dioxide by sol-gel method for magnetic applications. Process Appl Ceram. 2015;9(1):43-51. [CrossRef] | [Google Scholar]
- Tasnim NT, Ferdous N, Rumon MdMH, Shakil MS. The Promise of Metal-Doped Iron Oxide Nanoparticles as Antimicrobial Agent. ACS Omega. 2024;9(1):16-32. [CrossRef] | [Google Scholar]
- Zheng Y, Xu B, Chen S. Population Pharmacokinetic Modeling Using Polymyxin B Free Plasma Concentrations from Published Reports and Evaluation of Dosage Regimens Based on Monte Carlo Simulation in Critically Ill Patients. J Clin Pharmacol. 2023;63(9):1036-1044. [CrossRef] | [Google Scholar]
- La Russa M, Macchia A, Ruffolo S. The Antibacterial activity of doped TiO2 for preventing biodeterioration on cultural heritage building materials. Int Biodeterior Biodegrad. 2014:96 [CrossRef] | [Google Scholar]
- Khan A, Hanson B, Dinh A. 705. Array. 2018;5(suppl_1) [CrossRef] | [Google Scholar]
- Haque MM, Khan A, Umar K. Synthesis, Characterization and Photocatalytic Activity of Visible Light Induced Ni-Doped TiO2. Energy Environ Focus. 2013;2(1):73-8. [CrossRef] | [Google Scholar]
- Shang C, Bu J, Song C. Preparation, Antimicrobial Properties under Different Light Sources, Mechanisms and Applications of TiO2: A Review. Materials. 2022;15(17):5820 [CrossRef] | [Google Scholar]
- Vershney R, Chelaramani K, Bhardwaj A, Siddiqui N, Verma SK. Synthesis Photocatalytic and Antibacterial Activities of Nickle Doped TiO2 Nanoparticles. Orient J Chem. 2018;34(6):3140-4. [CrossRef] | [Google Scholar]
- Fan X, Zhu S, Wei X. Preparation and properties of antibacterial biomaterial PAA/TiO2/Ag. Polym Compos. 2023;44(9):5769-80. [CrossRef] | [Google Scholar]
- Wani PN, Kale SK, Dahiwale SS. Ion beam irradiation: Novel approach for preparation of Ag coated N doped nanocrystalline anatase TiO2 films. Mater Sci Eng B. 2023;288:116212 [CrossRef] | [Google Scholar]
- Mohammed BK, Zamel RS, Khlaief JH. Effect of Silver Variation on Ag-TiO2 Nanocomposite Synthesized by Sol-Gel. In Review. 2022 [CrossRef] | [Google Scholar]
- Liao C, Li Y, Tjong SC. Visible-Light Active Titanium Dioxide Nanomaterials with Bactericidal Properties. Nanomaterials. 2020;10(1):124 [CrossRef] | [Google Scholar]
- Kedziora A, Strek W, Kepinski L, Bugla-Ploskonska G, Doroszkiewicz W. Synthesis and antibacterial activity of novel titanium dioxide doped with silver. J Sol-Gel Sci Technol. 2012;62(1):79-86. [CrossRef] | [Google Scholar]
- Miditana SR, Tirukkovalluri SR, Raju IM. Synthesis and antibacterial activity of transition metal (Ni/Mn) co-doped TiO2 nanophotocatalyst on different pathogens under visible light irradiation. Nanosyst Phys Chem Math. 2022;13(1):104-14. [CrossRef] | [Google Scholar]
- Ahmad A, Thiel J, Shah SI. Structural effects of niobium and silver doping on titanium dioxide nanoparticles. J Phys Conf Ser. 2007;61(1):11 [CrossRef] | [Google Scholar]
- Cockerill FR. Methods for Dilution Antimicrobial Susceptibility Tests for Bacteria That Grow Aerobically: Approved Standard – Ninth Edition. 2012 [CrossRef] | [Google Scholar]
- Shi H, Magaye R, Castranova V, Zhao J. Titanium dioxide nanoparticles: a review of current toxicological data. Part Fibre Toxicol. 2013;10(1):15 [CrossRef] | [Google Scholar]
- Ziental D, Czarczynska-Goslinska B, Mlynarczyk DT. Titanium Dioxide Nanoparticles: Prospects and Applications in Medicine. Nanomater Basel Switz. 2020;10(2):387 [CrossRef] | [Google Scholar]
- Heydari Z, Ghadam P. IOCN 2023. 2023:43 [CrossRef] | [Google Scholar]
- Acosta S, Borrero-González LJ, Umek P, Nunes LAO, Guttmann P, Bittencourt C, et al. Nd3+- Doped TiO2 Nanoparticles as Nanothermometer: High Sensitivity in Temperature Evaluation inside Biological Windows. Sensors. 2021;21(16):5306 [CrossRef] | [Google Scholar]
- Javed R, Ain NU, Gul A. Diverse biotechnological applications of multifunctional titanium dioxide nanoparticles: An up-to-date review. IET Nanobiotechnol. 2022;16(5):171-89. [CrossRef] | [Google Scholar]
- Silva WS, Silva ACA, Rocha U, Dantas NO, Silva WF, Jacinto C, et al. Nd3+ doped TiO2 nanocrystals as self-referenced optical nanothermometer operating within the biological windows. Sens Actuators Phys. 2021;317:112445 [CrossRef] | [Google Scholar]
- . Nanooptics and Photonics, Nanochemistry and Nanobiotechnology and Their Applications. 2021:153-64. [CrossRef] | [Google Scholar]
- Ahmad MA, Yuesuo Y, Ao Q, Adeel M, Hui ZY, Javed R, et al. Appraisal of Comparative Therapeutic Potential of Undoped and Nitrogen-Doped Titanium Dioxide Nanoparticles. Molecules. 2019;24(21):3916 [CrossRef] | [Google Scholar]
- Gui DY, Ma XY, Yuan HD, Wang CH. Mn- and Yb-Doped BaTiO3-(Na0.5Bi0.5)TiO3 Ferroelectric Relaxor with Low Dielectric Loss. Materials. 2023;16(6):2229 [CrossRef] | [Google Scholar]
- Dubey V, Kaur J, Agrawal S. Synthesis and characterization of Eu3+-doped Y2O3 phosphor. Res Chem Intermed. 2015;41(1):401-8. [CrossRef] | [Google Scholar]
- Alshoaibi A, Kanoun MB, Ul Haq B, AlFaify S, Goumri-Said S. Insights into the Impact of Yttrium Doping at the Ba and Ti Sites of BaTiO3 on the Electronic Structures and Optical Properties: A First-Principles Study. ACS Omega. 2020;5(25):15502-15509. [CrossRef] | [Google Scholar]
- He C, An Y, Deng C. Tunable photoluminescent properties of rare-earth co-doped (Na0.5Bi0.5) TiO3 ceramics by Pr3+ concentration. Mod Phys Lett B. 2019;33(26):1950323 [CrossRef] | [Google Scholar]
- Gunasekaran A, Rajamani AK, Masilamani C, Chinnappan I, Ramamoorthy U, Kaviyarasu K, et al. Synthesis and Characterization of ZnO Doped TiO2 Nanocomposites for Their Potential Photocatalytic and Antimicrobial Applications. Catalysts. 2023;13(2):215 [CrossRef] | [Google Scholar]
- Rajput P, Deshpande MP, Bhoi HR. Photocatalytic and antibacterial activity of Yttrium doped TiO2 nanostructure. Chem Phys Impact. 2022;5:100101 [CrossRef] | [Google Scholar]
- S Ayn SN, Sulistiyani I, Nurdin M, Salim LOA. Metal-doped TiO2 photocatalyst as antibacterial-A Review. Res J Chem Environ. 2022;25(8):129-35. [CrossRef] | [Google Scholar]
- Cotolan N, Rak M, Bele M, Cör A, Muresan LM, Milošev I, et al. Sol-gel synthesis, characterization and properties of TiO2 and Ag-TiO2 coatings on titanium substrate. Surf Coat Technol. 2016;307:790-9. [CrossRef] | [Google Scholar]
- Han B, Pei Z, Shi L. TiO2 Nanoparticles Caused DNA Damage in Lung and Extra-Pulmonary Organs Through ROS-Activated FOXO3a Signaling Pathway After Intratracheal Administration in Rats. Int J Nanomedicine. 2020;15:6279-94. [CrossRef] | [Google Scholar]
- Dyshlyuk L, Babich O, Ivanova S. Antimicrobial potential of ZnO, TiO2 and SiO2 nanoparticles in protecting building materials from biodegradation. Int Biodeterior Biodegrad. 2020;146:104821 [CrossRef] | [Google Scholar]
- Wojciechowska A, Markowska-Szczupak A, Lendzion-Bieluń Z. TiO2-Modified Magnetic Nanoparticles (FeO) with Antibacterial Properties. Mater Basel Switz. 2022;15(5):1863 [CrossRef] | [Google Scholar]
- Page K, Palgrave RG, Parkin IP, Wilson M, Savin SLP, Chadwick AV, et al. Titania and silver-titania composite films on glass-potent antimicrobial coatings. J Mater Chem. 2006;17(1):95-104. [CrossRef] | [Google Scholar]
- Wang S, Ni Y, Wang J. Construction of ROS-generation system based on lipid droplet-targeting photosensitizer to mediate multiple subcellular organelle damage. Sens Actuators B Chem. 2023;393:134201 [CrossRef] | [Google Scholar]
- Wu Y, Liu R, Liu J, Jia J, Zhou H, Yan B, et al. The role of crystallinity of palladium nanocrystals in ROS generation and cytotoxicity induction. Nanoscale. 2023;15(13):6295-305. [CrossRef] | [Google Scholar]
- Ling P, Yang P, Gao X, Sun X, Gao F. ROS generation strategy based on biomimetic nanosheets by self-assembly of nanozymes. J Mater Chem B. 2022;10(46):9607-12. [CrossRef] | [Google Scholar]
- Fujishima A, Zhang X, Tryk D. TiO2 photocatalysis and related surface phenomena. Surf Sci Rep. 2008;63(12):515-82. [CrossRef] | [Google Scholar]
- Etacheri V, Di Valentin C, Schneider J, Bahnemann D, Pillai SC. Visible-light activation of TiO2 photocatalysts: Advances in theory and experiments. J Photochem Photobiol C Photochem Rev. 2015;25:1-29. [CrossRef] | [Google Scholar]
- Li Q, Mahendra S, Lyon DY. Antimicrobial nanomaterials for water disinfection and microbial control: Potential applications and implications. Water Res. 2008;42(18):4591-602. [CrossRef] | [Google Scholar]