ABSTRACT
As the first monoclonal antibodies [mAbs] were produced in 1975, the challenge to improve antibody engineering started. Since then, therapeutic antibodies have become the predominant class of new drugs developed recently, and an essential part of progress has affected cell lines. From the first pioneer hybridoma cells to the current vanguard Chinese Hamster Ovary [CHO] cells dramatic improvement has been seen. An essential part of this process is choosing the suitable cell line to seed the targeted antibody gene. This review encompasses all the current evidence to compare various proper cell lines for monoclonal antibody production such as mammalian cells, plant cells, bacterial cells, and yeast cells. Valid long-term data, regarding glycosylation, efficiency, and safety, support the current popularity of CHO cells. At the same time, other types of cell lines also show some promise for emerging needs for more therapeutic antibodies on the market.
INTRODUCTION
Since 1975, when César Milstein and Georges Köhler introduced antibody-secreting by hybridoma cells, monoclonal antibody technology has been progressively applied to a wide variety of biological and medical issues of both theoretical and practical concerns which nowadays they have become the dominant type of biopharmaceutical.1,2 Since their introduction, mAbs have been applied to treat autoimmune disorders, allergic diseases, transplantation rejection, anti-idiotype vaccines, and cancer.3,4 Scientists have developed complete mAbs with the same immunogenic properties as human Immunoglobulin [Ig] from endogenous animals.2 They have combined phage display technology with transgenic mice that express the human variable domain. The first therapeutic monoclonal antibody, muromonab-CD3 [Orthoclone OKT3] made by Johnson and Johnson, received the US Food and Drug Administration [FDA] approval in 1986. This mAbs was created from a murine monoclonal antibody [mAb] against CD3 expressed on T cells, which was utilized to treat acute transplant rejection by acting as an immunosuppressant.5,6 Because of the enormous rise in the previous several years, the FDA has authorized 79 therapeutic mAbs for numerous clinical applications, including infectious illnesses, cancer, immunological disorders, and arthritis.5 This growth showed a 51% rise in the number of mAbs or conjugates between 2014 and 2019, and there is still significant growth potential in this area.5–7
In recent years, mAbs have become one of the most highly demanded classes of biological products with high efficiency in targeted therapies with raised specificity, potency, and low toxicity quality.2 They account for nearly half of the market and are among the top five therapeutic proteins consumed in recent years. By 2024, the mAb market is predicted to reach $138.6 billion.8
Despite their impressive performance, mAbs still hold numerous disadvantages, including instability, high-dose prescription, and poor compliance.2
Antibody Structure and Function
An antibody is a large immunoglobulin glycoprotein, which recognizes foreign antigens, neutralizes them, and triggers additional immune responses.9 Antibodies have a fundamental structure that includes a constant Fc [crystallizable fragment] domain and an antigen-binding domain comprised of the Fv [variable fragment] and Fab region [antibody binding fragment] [Figure 1].9,10
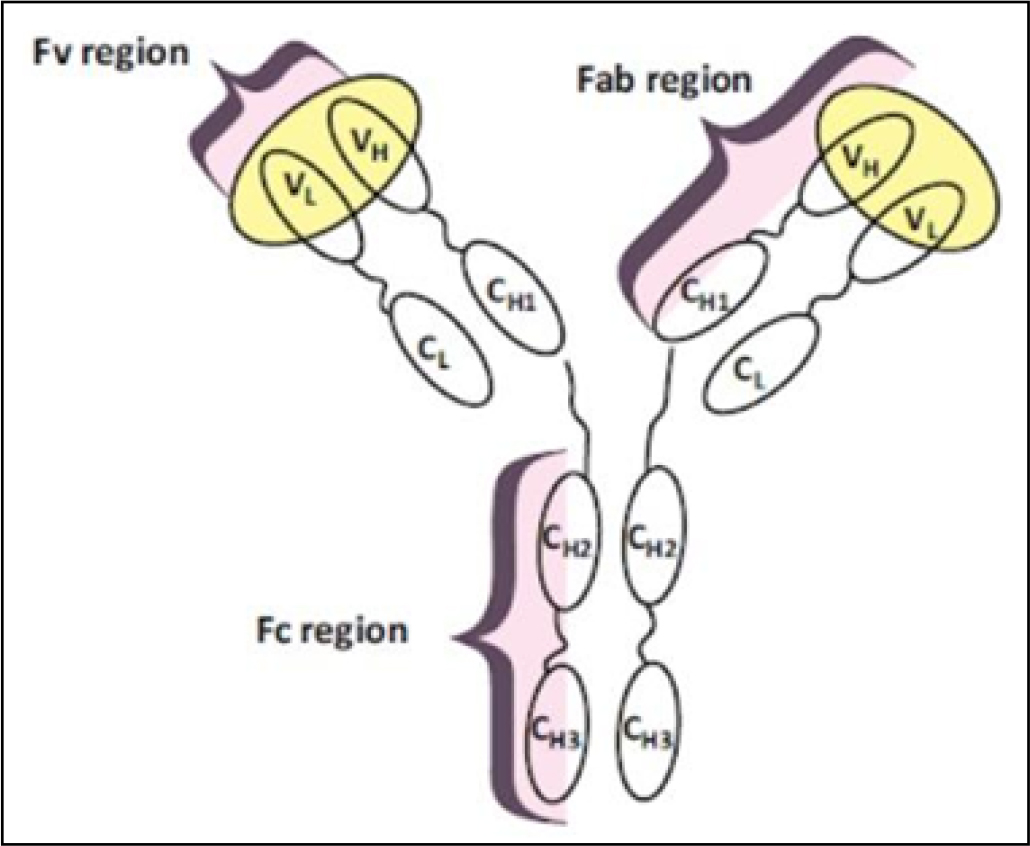
Figure 1:
Diagram of a complete antibody [yellow highlights indicate where the antigen binds].10
Fc regions are part of antibodies which are called heavy chains [Figure 2].12 Based on their heavy chains, antibodies are classified into five types: IgA, IgE, IgD, IgM, and IgG. Fc regions of antibodies are identified by Fc receptors [FcRs]. These receptors are positioned in several classes of immune cells, such as neutrophils, Natural Killer [NK] cells, eosinophils, dendritic cells, and monocytes.11
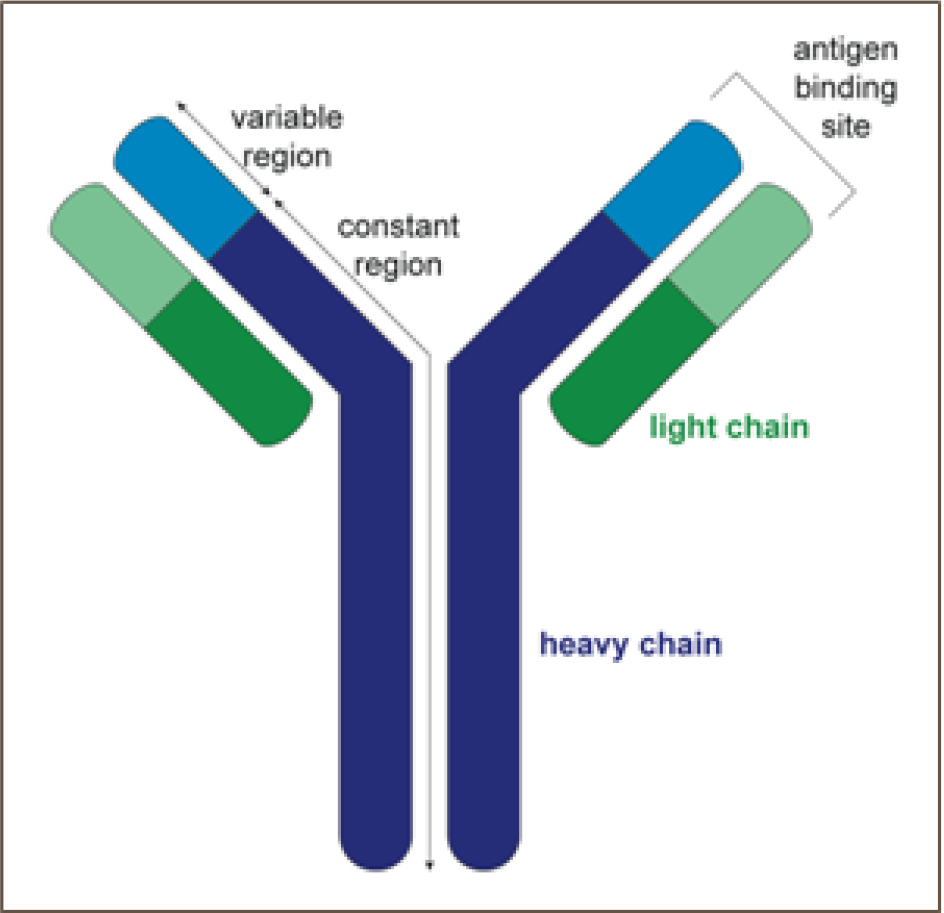
Figure 2:
A total antibody diagram demonstrating heavy and light chains.12
As an example of function antibodies, IgG could be utilized to treat Complement-Dependent Cytotoxicity [CDC], along with Antibody-Dependent Cellular Cytotoxicity [ADCC]. There are many subgroups within the IgG class based on the capacity of the Fc region to enable ADCC and CDC: IgG3 and IgG1 can cause ADCC and CDC, but IgG2 and IgG4 cannot. A monoclonal antibody is a large-scale product form of an antibody isotype that specifically targets an antigen epitope.11
The term monoclonal antibody [mAb] refers to antibodies that recognize only one epitope. In the human immune system, cloned homogeneous hybrid cells [B cells] make these antibodies.13 Native full-length mAbs are glycosylated during production. While not directly interfering with antigen function, the glycosylated Fc domain stabilizes antibodies and is required for ADCC. Additionally, glycosylation affects the pace at which recombinant mAbs are cleared from the body, and incompatible glycoforms might produce severe immunogenic consequences in patients. A full-length antibody does not need to have a glycosylated Fc domain in order to identify an antigen. The Fv and Fab regions [Figure 1] contain antigen-binding capabilities. Antibody fragments penetrate deeper into tissues and have a shorter retention duration in non-target tissues than mAbs. While the absence of the product’s stabilizing Fc domain makes it less stable, the lack of glycosylation on both the Fv and Fab regions makes it easier to construct and culture microbial hosts such as bacteria and yeasts.9,10
Researchers have discovered the method through which antibodies destroy tumor cells by providing efficient, consistent, and long-lasting cancer-suppressive effects in experimental and clinical tests. In reality, the appearance of spontaneous or increased tumor cells in the body triggers antitumor responses. A high number of antitumor antibodies are generated as part of this antitumor response, which directly destroys tumor cells while also damaging vascular and stromal cells. Scientists have investigated methods to create mAbs that selectively target a certain antigen present on the surface of cancer cells to increase mAb activity encouraging such anticancer processes. Every mAb targets a distinct malignant cells antigen, and their actions vary based on the antigenic targets of the various kinds of cancer cells. There are three categories of mAbs in general. A variety of methods are used by these agents, including stimulating the immune system to fight cancer cells, reducing the number of cancer cells that divide, and delivering chemotherapeutic medications or radiation directly to these cells.14
When changes in the manufacture of mAbs occur, biopharmaceutical firms are becoming increasingly interested in creative strategies to supply new protein therapy options. Since antibody treatments require big dosages over a long period of time, the manufacture of large quantities of antibody medications with cost and time efficiency is required to fulfill clinical standards and progress toward commercialization. Pharmaceutical firms strive to minimize time-to-market, keep costs low, and enable production flexibility while preserving desired quality qualities.15 One of these approaches is to find and use an appropriate cell line for the synthesis of mAbs. The sections that follow will look at current cell lines, vectors, and the best culture types for producing stable mAbs.
Expression systems
To produce mAbs, a suitable cell line must be selected, depending on the type of cell.4
Initially, cell lines used to be selected by their growth patterns and morphologies; yet, as culture conditions and time passed, these characteristics may change. There is an even greater need to identify established cell lines by additional criteria, given the growing number of cell lines.16
Chosen cell lines are expected to have some of the properties that are based on stoichiometric and kinetic parameters; such as high growth rates, the ability to produce high levels of product over a long period while maintaining cell concentration, along with additional characteristics such as cell line stability, virus vulnerability, and be able to process protein after translation and also, the obtained products do not endanger human health. When selecting the suitable cell for the possible cell line, it is important to optimize the medium composition and bioreactor operating conditions for improving specific productivity per cell.15 In addition to the aforementioned features, there are no constraints on employed cell lines, and the most suited cell line product should be chosen.4,16
Mammalian cells have become more vital in the current biotechnology industry because of their capacity to conduct [human-like] post-translational alterations, as indicated by their substantial market share and the importance of proteins obtained from this technique.16 In addition to prokaryotic [insect and plant cells] and eukaryotic [insect and plant cells] systems, mammalian cell lines are the leading system used to produce pharmaceutical proteins, including mAbs.4
Developing production culture processes and reforming cell lines allowed biopharmaceutical companies to achieve recombinant antibody titers up to 3–8 g/L for fed-batch mammalian cultures over 10 kilos.17
Mammalian cell lines
Many expression techniques were used to create antibodies and antibody-based compounds. African green monkey kidney cells [COS], a mammalian cell line, are an acceptable alternative for producing modest amounts of mAbs for biochemical and biophysical study.4 They are, however, not the best cells for large-scale industrial operations since their capacity to produce diminishes with time. Cell lines from mammals Because of their ability to perform complex posttranslational modifications, which result in the production of proteins similar to those found naturally in humans, Chinese Hamster Ovary [CHO] cells and murine myeloma cells are the dominant hosts for the commercial production of therapeutic glycoproteins and non-human glycan structures.18–20
Chinese Hamster Ovary [CHO] cells
Several industrial-scale recombinant proteins are synthesized utilizing Chinese Hamster Ovary [CHO] cells.17 The fact that this sort of cell line has been well-defined and effectively employed for the synthesis of a variety of therapeutic biopharmaceuticals implies that this trend will continue.21
According to a survey conducted in 2013 in the US or EU, most mAbs are produced in mammalian cells. The reliance on deliveries is increasing as more progress is being made in this area.22
Theodore Puck isolated the first CHO cells from Chinese hamster ovary tissue in 1957. The CHO-ori cells developed from Chinese hamster ovary cells isolated from these animals were found to be immortal. In 1968 following clonings of the CHO-ori cell line made CHO-K1 cell lines possible. Similar to CHO-ori cell lines this new cell line had limited advantages for large-scale processes and also they both were grown in adherent cell culture conditions. Thompson developed CHO-S, a subline that could grow in suspension culture. In 1971. Using this breakthrough of making CHO cells suspension-compatible, researchers were able to grow this cell line in bioreactors at a much larger scale.23 Since then their population as the dominant cell line has increased due to their easy culturability, and rapid proliferation and they show tolerance to chemically-defined media. It is also a positive characteristic of CHO cells that the downstream purification process is facilitated by the lack of replication of HIV, influenza, and polioviruses. Mentioned features combined with the fact that they typically don’t produce variable outcomes enhance the reliability of mAbs. Furthermore, CHO cells are easily genetically modified, making them an ideal choice for producing mAbs.22
The regulatory approval procedure for CHO cells is less difficult due to their capacity to create safe, biocompatible, and bioactive mAbs, and they are governed by their Product Quality Attributes [PQAs]. Product quality features include charge variations, glycosylation, oxidation variants, measure variants, and structural variances. The main criteria defining mAb quality include potency, Pharmacokinetics/Pharmacodynamics [PKPD], immunogenicity, and product safety.21–24
In clonal selection, MTX [methotrexate] injection is utilized to select cells that express sufficient amounts of DHFR [dihydrofolate reductase] because recombinant CHO cell lines produce DHFR enzymes that are resistant to MTX effects. Due to the fact that CHO cell lines can produce large amounts of recombinant protein [like mAbs] when Methotrexate [MTX], 15 is used as an inhibitor, they have been amplified together with the Dihydrofolate Reductase [DHFR] gene as well as the intended recombinant gene.25 It is understood that there is a possibility of gaining resistance to MTX by amplifying the DHFR gene. A significant difference exists between the amplification units of gene-specific expression vectors and those of the DHFR gene, owing to the larger gene-specific expression vectors [100–3,000 kilobases [kb]] that co-amplify genes of interest that are adjacent to the host genome. High gene copy numbers may not be enough to boost productivity in highly amplified subclones due to transcriptional and post-transcriptional limitations, even though the level of gene amplification usually correlates with the level of expression. As a result of high levels of amplification and expression, foreign gene products can exert a variety of levels of toxicity and metabolic burden on cells. Since cells regulate gene expression in response to stress, this may lead to instabilities in recombinant protein production. Recombinant proteins can modulate any stage of gene expression, including transcription, which is considered to be the primary determinant of protein expression.26
Several studies have been conducted on genetically engineering the production of host cells to improve or modify product quality or host cell strength. In recent years, glycosylation of antibodies gained much attention, which involves adding glycan structures to products, thereby affecting their clearance rate and bioactivity.15 Monitoring and controlling qualities such as mAbs N-linked glycosylation of the Fc domains27 are vital because of their effects on PK/PD [Pharmacokinetics and Pharmacodynamics], efficacy, and immunogenicity. In addition to immunogenicity, glycan species differ in relative abundance, affecting their circulation half-time, making glycan species potential CQAs [Critical Quality Attributes]. As a result, glycosylation affects galactosylation levels, which in turn can reduce Complement-Dependent Cytotoxicity [CDC]. On the other hand, Despite maintaining FcRn binding and in vivo half-life, non-glycosylated mAbs have minimal effector activity. However, some non-human glycosylation substrates, such as N-glycolylneuraminic acid and α-galactosyl epitopes, may induce immune responses. The N-linked glycosylation in the Fab domain of Cetuximab28 has caused anaphylaxis in some individuals since Some patients have IgE antibodies to α-galactosyl-containing N-linked glycoproteins on the Fab.27
Furthermore, core-fucosylation levels are also reduced, which leads to increased Antibody-Dependent Cytotoxicity [ADCC]. And finally, The immunogenicity potential of mAbs was improved by inserting non-human epitopes such as galactose-alpha- 1,3-galactose residues and N-glycolylneuraminic acid residues in the glycosylation process.24
Glycoform profiles of mAbs are under influence of several factors, including the type of cell line used, the media composition, feeding strategy, and process parameters during cell culture. Glycosylation machinery regulatory mechanisms, which depend on the cell line and protein sequence and structure, are a factor counting as inconsistency on the universal scale. Despite this, some qualitative trends have been observed consistently across many studies.24,27,29
According to these study results, the proportion of mature glycoforms declines during the culture. The culture advances by increasing galactosylation and underprocessed glycoforms, such as high-mannose species. Sugar nucleotide levels, glycosylation enzyme levels, and cofactors like metal ions can all impact the timing of glycosylation network activity.30 During the culture, significant changes in gene expression profiles are also observed in the cell cycle. Throughout the culture, the specific productivity [QP] of the mAb generally shows changes.31
Despite the absence of rigorous investigation to support the assumption that transfection into a similar host cell might lead to significant clone-to-clone variance, it is widely accepted that glycosylation is dictated by the host cell utilized in cell-line formation. When manufacturing scales up, it is also vital to maintain constant N-linked glycosylation throughout batches and large-scale bioreactors. As previously stated, several variables, such as cell culture and/or processing settings, might impact glycosylation. As a result, companies have begun incorporating N-linked glycosylation profiling into their cell-line development processes, forcing them to choose the final production of cell lines based on another factor known as optimal N-linked glycosylation profile, in addition to the frequently mentioned production yield, intrinsic efficiency, and optimized cell growth conditions.27
Murine cells
Another frequently used cell line for large-scale mAbs is Murine cells [e.g., NS0, Sp2/0-Ag14]. NS0 and Sp2/0-Ag14 cells are repeatedly cloned and chosen. From BALB/c mouse plasmacytoma cells, immortalized B cells that do not release Immunoglobulin G are generated [IgG]. To establish these two parental cell lines, the original cell line was cloned many times and, in the case of Sp2/0-Ag14, fused with spleen cells from another BALB/c animal.32,33 These cells are cholesterol auxotrophs, meaning they require cholesterol in their culture medium for growth.4,18,32 In 1962, Potter and Boyce realized that injecting mineral oil into the intraperitoneal cavity of BALB/c mice caused plasma-cell neoplasms.34,35 In later studies, Potter and Boyce discovered that one of the tumor-induced inbred BALB/c female mice was able to secrete IgG1. Horibata and Harris created a cell line from these tumor cells in 1970. Galfre and Milstein created NS0/1, a non-secreting cell line, after removing IgG1 synthesis from the cell line in 1981. Since then, NS0 has been used to generate mAbs for a range of disorders.22
To lessen the risk of Transmissible Spongiform Encephalopathies [TSE], producers have employed sheep wool-derived cholesterol instead of bovine traces on a large scale. Apart from animal-derived cholesterols, synthetic cholesterol solutions such as SynthecolTM [Sigma-Aldrich, St. Louis, MO, USA] and lipid concentrate [Life Technologies, Grand Island, NY, USA] are available on the market. A recent study found that electrohydrodynamic spraying cholesterol nanoparticles into NS0 cells lowered toxicity compared to synthetic cholesterol solutions.32
The NS0 cells are characterized by the absence of Glutamine Synthetase [GS] enzyme activity.32,33 This feature can be employed as a marker for recombinant antibody expression. Nonetheless, Non-GS NS0 cell lines have also been reported to produce antibodies up to 3 g/L.35 One of the advantages of Sp2/0-Ag14 in contrast to NS0 cells is they experience several spontaneous mutations which make them needless to satisfy glutamine requirements.33
A disadvantage of Mouse-derived cell lines, including NS0, is the production of N-glycosyl Neuraminic Acid [NGNA], a sialic acid that is not found in human antibodies and might have immunogenic properties. This immunogenicity concern might partially explain why NS0 cells are not used as widely for producing therapeutic antibodies as CHO cells.15 α-gal and Neu5Gc are the other immunogenic products of murine cells which they produce at a noticeable amount which is another disadvantage of these cells in contrast to hamster cells.35
Antibodies are frequently produced using the host cell lines NS0 and Sp2/0-Ag14. Cetuximab [ERBITUX®] and palivizumab [SYNAGIS®] are some of the well-known mAbs produced by utilizing these cell line systems.35
Due to the more extensive characterization of CHO cells from an ‘omics perspective, it might be worthwhile to continue studying and characterizing NS0 cells.22
Human cell lines
Despite the widespread use of CHO cells in the past few years and the availability of a wide range of mAbs derived from them, In most cases, human cells can produce proteins that are similar to those of humans. This may be a positive characteristic in comparison to other animal cell lines.36,37 Several products made from these human cell lines have been tested in large-scale clinical trials. Furthermore, these cell lines are useful as expression systems in biomedical research since they are high-yielding and contain the same [post-translational modifications] PTMs as normal human cells.37
In 1951 the first human cell line was created by isolating cervical cancer cells. In the 1960s, human diploid cells made vaccine production possible. Their acceptance, however, was held back because of concerns about latent oncogenic agents in these cell lines without even representative phenotypic characteristics.37 In 1973, human embryonic kidney cells [HEK] were transformed with adenovirus type 5 [Ad5] sheared pieces of DNA, which led to the immortalization of the cells in the form of the HEK293 line. As a result of the adenovirus’s integration, the Ad5 genome is believed to have been merged with the 19th chromosome of these cells. Many development characterizations have been done on this type of cell line, which led to the development of numerous subtypes of the cell line, as mentioned previously.38 Because of their rapid growth, high protein output, and investment in system optimization, human cell lines are now commonly employed in the production of viral vaccines. They also serve as a substrate option for the synthesis of recombinant proteins and mAbs instead of CHO cells.37
Various types of human cells are used as cell lines. For instance, the HEK293 cell line which originated from epithelial cells, the HT-1080, produced from an epithelial-like phenotypic fibrosarcoma, and the PER. C6 cell line which is originating from immortalized with adenovirus E1 gene transfection human embryonic retinal cells.37,38 Nonetheless, being widely used, the HEK293 cell line was more in the attention of researchers, leading to many derivative cell lines which can produce a wide range of recombinant proteins and mAbs.38 These cell lines can produce with high productivity, and their products are approved as recombinant biotherapeutic products. more are being considered and tested.37,39
The production of biotherapeutic proteins, such as mAbs, relies almost exclusively on HT-1080 and HEK293 cell lines.37 As an advantage of these cells, both HEK293 and HT-1080 are capable of passing their products through human PTM.40
HEK cells were initially modified to produce in small-scale quantities such as research amount protein productions. But as their potential to get transfected was realized, they became widely popular in large-scale production, and HEK293 became the predominant cell line today.41 Aside from PTM, which these cells accomplish considerably more promising than the CHO cell line and the murine myeloma NSO cell line, these cells can grow in serum-free suspension cultures and have transfecting capacities.38,42,43 This cell line’s performance has been altered by a combination of these traits and genetic alterations across the biological process, including cell growth, apoptosis, metabolism, glycosylation, release, and protein folding, as well as bioprocess, medium, and vector optimization.38
Inefficient glucose metabolism is a major disadvantage of HEK293, pushing the cell to operate predominantly on glutamine for energy generation. Due to the use of glutamine as an energy source, ammonia and lactate are formed as byproducts. These by-products are toxic to premature cells, which causes their death. Furthermore, high ammonia levels disrupt the glycosylation of proteins. To improve this issue, researchers on HEK293 tend to enhance and reformulate central carbon metabolism. When the glycolysis and tricarboxylic acid cycle were restored by overexpressing the Pyruvate Carboxylase gene [PC] and removing the Pyruvate Dehydrogenase gene [PDK], as well as its activator, the Hypoxia-Inducible Factor 1 gene [HIF1], the cell density increased, as well as lactate and ammonia production decreased. Additionally, these modifications improved recombinant protein expressions and glycosylation, mixed amino acid usage, and enhanced recombinant viral titers by 30 times.38
Human cell lines are progressively being used to manufacture biotherapeutic proteins; some products have already been authorized for clinical use, and others are in the works. In this method, immunogenic proteins can be produced using human expression systems rather than possibly immunogenic non-human PTMs [such as a-gal, NGNA, and others]. With increased research funding and further technical improvements, human cell lines may be used to generate proteins more successfully in the future. Human cell lines are projected to improve as more advanced collecting technologies are developed, and these cell lines will become one of the most desired platforms for generating protein biotherapeutics such as mAbs.37
Plant cell lines
A popular alternative to animal-based recombinant protein and antibody production is molecular farming, using plants to produce recombinant proteins and antibodies at scale.44,45 Plant cells/tissues are being cultivated in vitro, or complete plants can be employed in Molecular farming for protein synthesis.46 These molecular farms can engineer tailored antibodies and glycosylation profiles and do not pose a risk to humans; they are a practical expression system.57 This sort of cell line is especially appealing owing to its lower costs and competitiveness, which can be achieved by simply increasing the number of plants.44,48
As noted before, plant cells aren’t as high-end and expensive as the CHO cell line. This option makes them an ideal candidate for alternative production platforms that could at least support currently over-exploited mammalian fermentation platforms. Since the plant cell line is considered to be reliable in aspects such as safety, scalability, rapidity, and lower manufacturing costs, this presents an opportunity.47,49
While bearing the advantages of being easily scalable in a whole-plant platform, this system has some disadvantages, such as the length of time it takes to develop stable transgenics, instabilities, and quality of the products, possible contamination of fertilizer, pests, and diseases potentially impacting the “production factory”, and the inability to integrate good manufacturing practice into the whole-plant process. Additionally, plant cell suspension cultures provide advantages over both microbial and animal cell culture systems, making them an advantageous method for developing pharmaceutical proteins.46
As a result of the pioneering studies that found plants could produce full-size IgG, plant cells are now commonly used to construct IgAs, Fab fragments, single-chain antibody fragments [scFvs], therapeutic monoclonals [mAbs], among other biopharmaceuticals.47–50
A majority of these pioneering studies used tobacco as a transgenic host to synthesize plant-derived recombinant proteins from leaf samples. Among the favorable expression hosts, they have advantages such as efficient expression with a rich transformation process potential, high biomass products, trouble-free scalability, and no contamination of the human and animal food chain since these yields are Inedible by humans or animals. These advantages make tobacco hosts a valuable asset in commercial molecular farming in several biotech companies.51
With all these advantages, one of the main downsides of tobacco products is they are highly contaminated with nicotine and other toxic alkaloids. Even though the high amount of these residues is withdrawn, the pharmaceutical industry has turned its attention to other leafy crops including lettuce, and Alfalfa. These leafy crops have been tested in clinical trials for the hepatitis B virus subunit vaccine.51,52
Medicago Inc., a Canadian biotech firm, is developing alfalfa cell culture and transient-expression technology to create high-level protein expression in alfalfa leaves. In addition to possessing homogenous glycan structures, Alfalfa leaves generate glycoproteins that are desirable for pharmaceutical synthesis. Another benefit of Alfalfa is a perennial that fixes nitrogen in the soil and has a high biomass output. Despite this, alfalfa is a feed crop with high levels of oxalic acid in its leaves, Which may impede harvest processing.51
In contrast to cereal seeds such as wheat, millet, rice, barley, or oats, leafy crops’ protein products tend to be unstable, so processing should be accomplished before degradation occurs. For this purpose, several studies have been done on cereals seeds, especially maize seeds. Among the advantages of maize seeds are their high biomass yield, their easy manipulation in vitro, and their easy scaling up in production. These benefits enable them to be employed on a commercial scale in the manufacture of technical proteins such as avidin and b-glucuronidase [GUS]. They are also being researched for vaccine subunits, recombinant antibodies, and aprotinin and laccase enzymes, which are also called Enzymes with technical properties.51
Because plants can be grown locally, they remove transportation issues and eliminate expiration problems, etc., so they are an option for vaccination campaigns. As a result, potato farms have been created to produce vaccines, they also have been used to produce antibodies and albumin Tumor Necrosis Factor-a [TNF-a].51,53
Developing a perfectly formed and functioning human antibody in transgenic tobacco plants provided evidence that plant-produced mammalian proteins would preserve their intended features.58 Using transgenic plants as a platform, bacteria were able to effectively create hepatitis B Virus-Like Particles [VLPs] as well as complex antibody products such as secretory immunoglobulin A [sIgA] and orally immunized proteins.54–56
Pharmaceutical production by plant cells has progressed from oral administration vaccines, the first generation of biopharmaceutical proteins produced by plants, to antibodies, subunit vaccines and VLPs, and therapeutic enzymes, as well as a diverse range of antibodies and antibody derivatives such as sIgA, IgM, and various IgG molecules.47
Protalix Biotherapeutics Inc. has developed reformed carrot cells to produce bio-pharmaceutical products commercially known as ELELYSO™ for treating Gaucher’s disease, a rare metabolic disease found in humans. following this massive success, Medicago Inc., for instance, created a quadrivalent seasonal influenza vaccine that just passed Phase III clinical trials [Efficacy, Safety, and Immunogenicity of a Plant-Derived Quadrivalent Virus-Like Particles Influenza Vaccination in Adults].49
Plant cells represent similar characteristics to both bacteria and mammalian cells in rapid doubling times and holding post-translational modification systems respectively. The similarities between them, as well as the fact that they don’t harbor any known human pathogens, allow them to be suitable for pharmaceutical protein production.46
An examination of the Post-Translational Modifications of Proteins in Plants reveals many similarities with those in the cell of animals. Plant proteins are folded and disulfide bonds are formed by chaperones, and cellular glycosyltransferases perform the addition of N-glycans.49
However, there are some differences between plant and mammal N-glycan structures, Specifically, the presence of extra sugars linked to the core. Plants glycans lack sialic acid residues in their structure, their biological properties may differ from those of mammalian glycans, which leaves plant glycans, with the core structure of a β[1,2]-xylose residue with an α[1,3]-fucose residue, which makes them recognizable as epitopes by allergen-reactive IgE.48
This type of cell line should be further researched and developed since plant cells could produce 10 mg/L proteins, which is not desirable as a commercial product. A profit margin of 5 to 10 times should be achieved by increasing this number. From gene expression through cell culture, process development, and protein purification, this manufacturing process must be changed at all stages. Similar to mammalian cell culture innovations that have resulted in today’s high levels of productivity, plant-based bioproduction of recombinant proteins will require these technologies.46
Fungi cell lines
Since mAbs and other therapeutic proteins became prominent as therapy choices, a tremendous amount of work has been put into decreasing development costs, cycle times, and, of course, meeting the rising demand for these products. Apart from CHO, yeast, and fungi are also being developed as alternative protein production systems.6 They are able to remain on the market because yeast systems have several benefits over mammalian cells, including rapid growth, easy gene management, simplicity of culture, steady expression, appropriate protein titers, rapid cell development, and low-cost scalable fermentation techniques.6,53
Except for all mentioned upper hands Yeast species give, their most important quality is eukaryote-specific post-translational modifications. This makes them highly useful for many applications in biotechnology, including protein production. Saccharomyces cerevisiae, Kluyveromyces Lactis, Hansenula polymorpha, and Pichia pastoris are examples of yeast species that have been developed over the last decade as host cells for production. In a well-known fed-batch fermentation technique, Pichia pastoris, a methylotrophic yeast, can grow to high cell densities making them capable of producing over 400 diverse proteins including human therapeutic proteins. Unlike mammalian cell cultures, Pichia pastoris cultures do not contain endotoxins or potential viral contamination.6
The glycosylation system utilized by mammalian cells differs greatly from that of yeast cells when it comes to producing therapeutic antibodies. Although yeast cells cannot express Fc regions and do not require glycosylation, yeast can provide an environment where various antibody fragments can be made [for instance, scFv, Fab, scAb, Db]. The production of glycoengineered yeast is proving to be successful due to the development of aglycosylated IgG variants comparable in effectiveness to glycosylated IgG without glycosylation. Current thinking suggests that yeast can be used to produce therapeutic antibodies.6,57
As mammalian cell antibody expression is reaching completion, attempts to improve antibody efficacy using glycoengineering are gaining momentum. Glycoengineered yeast has been demonstrated to improve antibody effector function by synthesizing mAbs with homogeneous N-linked glycans. As a robust and cost-effective alternative to mammalian cells, glycoengineered yeast expression platforms enable not only the explanation of structure-function links but also the research of protein structure-function correlations.58
The majority of glycoproteins expressed by plants result in oligosaccharides with xylose and fucose chains that are immunogenic to humans. A glycoengineered moss [Physcomitrella patens] has been developed by Greenovation Biotech [Heilbronn, Germany] that lacks xylose and fucose transferase activities. The Pittsburgh, NC-based biotech company Biolex Therapeutics has developed a method for inhibiting endogenous fucosyl and xylosyl RNA interference inhibits transferase activity employing engineered duckweed [Lemna minor].58
The reason why glycosylation is necessary is that The Fc region is recognized by Fc-binding ligands [FcgRs and C1q], and the activation of effector processes such as Antibody-Dependent Cell-mediated Cytotoxicity [ADCC], antibody-dependent cell-mediated phagocytosis [ADCP], and Complement-Dependent Cytotoxicity [CDC] all depend on glycosylation of Fc regions at the Asn297 position.57
It has already been stated that yeasts are valuable hosts due to their multiple advantages, such as obtaining posttranslational changes. Non-methylotrophs and methylotrophs are the two types of yeast expression systems.59
Saccharomyces cerevisiae
One of the first and most popular yeast expression systems starting from the 1980s is Saccharomyces cerevisiae which is used primarily in traditional brewing, winemaking, and baking.
S. cerevisiae is non-methylotrophic yeast whose heterologous hyper-mannosylated glycan structure glycoprotein products are low activity and high immunogenicity. Protein secretion increased after N-glycosylation modification was applied to S. cerevisiae. Intracellular expression of recombinant proteins is possible, as is targeting the secretory system with secretory signal peptides. This trait stimulates the use of Saccharomyces cerevisiae in numerous industrial processes since it is nonpathogenic and has traditionally been utilized, as previously noted, in various nutritional sectors and the manufacturing of biopharmaceuticals.
S. cerevisiae is now commercially producing Hepatitis B surface antigen, hirudin, insulin, glucagon, urate oxidase, macrophage colony-stimulating factor, and platelet-derived growth factor.59
Nevertheless, S. cerevisiae market products face several limiting factors, including hyperglycosylated proteins, low protein yields, and plasmid instability. As a result, alternative expression systems, such as methylotrophic yeasts Pichia pastoris and Hansenula polymorpha and non-methylotrophic yeasts Yarrowia lipolytica, Kluyveromyces lactis, and Arxula adeninivorans have been designed to express proteins.59
Pichia pastoris
As previously stated, using Pichia pastoris to create heterologous proteins such as industrial enzymes and biopharmaceuticals is one of the alternate approaches for recombinant protein production. Some of the products of this methylotrophic expression system include human erythropoietin, human monoclonal antibody 3H6 Fab fragment, human serum albumin, and collagen. With the advancement of genetic techniques and the availability of novel strains, P. pastoris has become the dominant producer of recombinant proteins. Because of its Potent methanol-regulated Alcohol Oxidase promoter [PAOX1], extremely effective secretion mechanism, and capacity to undergo post-translational modification, this host is also of industrial importance. Nevertheless, like Non-methylotrophic S. cerevisiae, Methylotrophic Yeast P. pastoris has several drawbacks that restrict its development capacity. These issues include excessive concentrations of protease, product-specific effects, control problems, and dangers associated with large methanol deposition. However, P. pastoris has been used to express a variety of recombinant proteins, including recombinant angiogenin. P. pastoris has also been shown to produce a nanobody [VHH] against Clostridium botulinum neurotoxin type E [BoNT/E], human adiponectin, recombinant xylanase, and anti-HIV antibody.59
In terms of economic issues, using experimental procedures to improve yeast cell culture parameters such as induction technique, pH, oxygen concentration, temperature, aeration, and oxygen density might give lucrative choices for boosting the function of this sort of cell line.60
For protein synthesis and antibody production, a wide range of vectors and host strains have been created, as well as a large range of markerless integration methods and quick deletion methods for yeast strains. Despite this, more optimization and reform are needed on the product of interest. In certain host strains like P. pastoris, glycosylation patterns that resemble those of humans must also be controlled. The homologous recombination feature of yeasts makes them a powerful tool for high-throughput analyses of drug screening and functional genomics to clone large pieces of DNA.59
Bacteria cell lines
Bacteria are crucial for the producers of recombinant proteins due to their capacity to grow at a dense low-cost culture, their well-understood genetics, and the number of cloning vectors and mutant host strains.61
As the industrial market for biopharmaceutical goods began in the 1980s, the FDA allowed the commercialization of humulin, a human insulin analog generated recombinantly in the bacteria E. coli. In addition to the first FDA-approved medicines, insulin, small, non-glycosylated proteins, have been delivered by selectable resistance markers, such as antibiotic resistance markers. As a result, an increasing number of New Biological Entities [NBEs] have been approved.61,62
Employing microorganisms in the manufacturing of biopharmaceuticals has several advantages. Microorganisms’ natural enzymatic machinery may create large molecular weight molecules such as proteins and perform extremely enantio- and regio-selective reactions. These goods emit no organic or inorganic pollutants like toluene or benzene. Moreover, microorganisms allow for the repeated application of immobilized enzymes or cells, resulting in lower overall expenses.61
As a result, numerous recombinant proteins, including as mAbs, interferons, and colony-stimulating growth factors, may be efficiently created using a bacterial expression plasmid that comprises selection markers, inducible promoters, Ribosome Binding Sites [RBS], and Multiple Cloning Sites [MCS]. Mature proteins frequently contain extracellular and periplasmic signal sequences to aid in their transport into separate compartments. By using recently modified vectors, proteins could be efficiently expressed and translocated into multiple compartments of bacterial cells.63,64
Escherichia coli
Escherichia coli [E. coli] is one of the most considerable and extensively used commercial expression systems for producing antibody fragments and mAbs in separate compartments of the bacterium. Since the licensing of humulin in 1982, the gram-negative bacteria E. coli has produced recombinant biopharmaceuticals such as antibodies [e.g., antitumor necrosis factor [TNF]-α Fab], accounting for roughly 40% of all commercialized biopharmaceutical drugs today. Antibodies are normally produced by bacteria using a self-replicating plasmid containing a ribosome binding site for the protein synthesis process and promoters from E. coli lactose operon [lac] and synthetic tryptophan operon [trp]. It is possible to enhance the quantity of biomass generated by E. coli by utilizing self-replicating multicopy plasmids; however, they impose a significant metabolic load on the organism, including cell growth inhibition and ultimately cell death. Due to the heavy metabolic burden imposed by self-replicating multicopy plasmids on bacteria, new plasmid-free expression systems have been developed that incorporate heterologous DNA into the chromosome in a site-directed manner to inhibit bacterial cell death.61,64
Since they manufacture their products in fragments, their products do not require post-translational changes such as glycosylation to become active. These antibody fragments are generated by E. coli cells in various compartments, as previously stated. They are produced intracellularly as Inclusion Bodies [IBs], periplasmically as soluble and active proteins, and extracellularly as secretory proteins via diverse secretory signals. Unlike a full antibody such as IgG, antibody fragments can be tiny, making them simpler to infiltrate tissues and ineffectual owing to a lack of constant domain [Fc]. It also has a shorter serum half-life and is less immunogenic than complete antibodies. Recent advances in simple and cost-effective large-scale manufacturing are attempting to aid in the successful expression and secretion of soluble and biologically active fragments into the extracellular space of E. coli in grams because the products in this path do not require troublesome refolding to become functional and cost-effective at large-scale manufacturing.63
Considering that E. coli could be genetically manipulated and its growth requirements are well understood, it remains the first choice and most commonly used prokaryotic host for non-glycosylated recombinant proteins and monoclonal antibody production. With molecular technologies, it is still feasible to influence E. coli production. Furthermore, it necessitates a simple and inexpensive substrate for proliferation and production, resulting in economically feasible technologies, lower Cost of Goods [COGs], and more affordable protein manufacturing.61,63,65
Manufacturing recombinant proteins in Escherichia coli, a gram-negative bacteria, is more efficient and cost-effective. Both heavy and light chain fragments are generated and transported from the cytoplasm to the periplasm using signal peptides derived from Outer membrane protein A [OmpA], alkaline Phosphatase A [Pho A], or Pectate lyase B [Pel B]. These routes transport proteins from the cytosol to the bacteria’s periplasmic region. The refolded scFv fragment was effectively produced in the periplasmic space of E. coli utilizing the pelB leader sequence and the flexible linker peptide [Gly4Ser1]3, which is beneficial for producing functional fusion proteins. This segment folds appropriately and becomes useful in the periplasm’s oxidizing environment.66 While Inclusion Bodies [IBs] are employed to make functional antibody fragments, this sort of reaction always produces less of them. Fab and scFvs were effectively generated by mutants and modified E. coli strains with glutathione or thioredoxin deficits after further optimization. Moreover, in E. coli bacteria, co-expression of the cytoplasmic molecular chaperones GroEL/ES, DnaK/J, DsbC, trigger factor, and Skp enhanced Fab synthesis in the cytoplasm.63 Apart from the approaches outlined above, a high yield may also be obtained by optimizing fermentation conditions so that recombinant protein synthesis includes a high cell density. For example, because the cell density in fermentation culture is nearly 100 times higher, hapten-specific scFv was generated at levels up to 1.2 g/L compared to 16.5 mg/L in shake flask culture.67
Currently, further approaches are being employed to produce these fragments in the E. coli system. In the first strategy, as small fragments of antibodies are expressed directly in the cytoplasm of E. coli without incorporating the desired signal sequences, the recombinant antibody fragments accumulate as insoluble IBs in the cytoplasm. To make a protein functional and active, in the final step, the insoluble IBs are treated in vitro with various chemicals and conditions for renaturation, folding, and disulfide bond formation.63
In an alternative pathway for producing antibody fragments, E. coli strains overexpressed with disulfide isomerase can be employed. Recombinant fragments formed from this enzyme form cytosolic disulfide bonds more efficiently. It is also possible to produce functional antibody fragments at high levels with FA113 E. coli, which provides an oxidizing environment. The production of functional antibodies can be achieved in two ways: 1) recombinant protein secretion into the periplasmic space or culture media, allowing protein folding and disulfide bond formation; 2) the collection of proteins in the cytosol as insoluble aggregates or IBs with the minimum amount of host-cell proteins in the resultant combination. Due to its high expression yield, All other techniques are preferable over cytoplasmic expression through insoluble complexes. This method, however, requires cost-intensive and labor-intensive refolding and purification steps. Despite all the efforts, many of the freshly expressed proteins are wasted, and only a tiny portion of the original protein is recovered.63
Unlike yeast and mammalian cells, this manufacturing system lacks a secretory mechanism. E. coli, on the other hand, employs a distinct secretion process to move expressed proteins from the cytoplasm to the periplasm.63,68
Recent studies have revealed that full-length glycosylated mAbs may be efficiently produced in E coli. The Campylobacter jejuni N-glycosylation mechanism and the possibility of importing it into E. coli might pave the way for the synthesis of glycosylated Fc domains and the successful expression of full-length mAbs in E. coli. As a result, pharmaceutical companies have prioritized the reintroduction of E. coli into their manufacturing facilities.61
One disadvantage of using recombinant proteins generated by E. coli for therapeutic purposes is that they must be purified in a second stage from endotoxins created by E. coli from Lipopolysaccharide [LPS]. These endotoxins are pyrogenic in humans and other animals, inducing an immunological response.61,63 Inclusion bodies form when there is an imbalance between folding helpers expressed inside the cell and disulfide bridges cannot form in the cytoplasm’s reductive environment. The answer is to synthesize the intended protein alongside chaperones or by fusing the destined protein to a leader peptide at the N terminus, which then delivers the protein to the periplasm.61 Additional alternatives may include Lowering the temperature, substitution of amino acids, co-expression of chaperones, hydrophilic extensive fusion partner, the addition of sorbitol, glycine-betaine, sucrose, and raffinose in the growth medium, changing culture conditions, pH, or changing the bacterial strain could all help to solubilize and refold these inclusion bodies and turn them into functional and active products.61
Second, E. coli has trouble manufacturing full-size antibodies with complex multimeric proteins composed of two Heavy Chains [HC] and two Light Chains [LC];63 also, the proteins generated in E. coli are inactive proteins that are aggregated or inactivated by nature. These proteins are glycosylated but not functional since they are misfolded.63,68 Although some protein folding occurs in E. coli periplasm.63
This led to the formation of new methods to achieve the purpose of monoclonal antibody production. Coexpression of molecular chaperones in oxidizing cellular environments, modification of protease-deficient strains, and the incorporation of expression-enhancing genetic elements are all methods of bridging disulfide bridges. In addition to selecting the correct vector for gene cloning, balancing the expression of heavy and light polypeptide chains, and optimizing the medium, feed, and culture conditions, genetically engineered E. coli cells were able to produce active Fab protein in the periplasmic space at a concentration of 2.0 to 5.0 g/L with the co-expression of isomerase DSbc.63
Furthermore, the E. coli cell line system is built on a simple, low-cost substrate for growth and production, making it a commercially viable product with a lower Cost of Goods [COGs] and the capacity to be manufactured at a lower cost. It can also be grown up and cultivated in large fermenters for higher protein production; moreover, the T7 inducible promoter’s flexibility may result in high-level gene and protein expression. Protein synthesis by E. coli in shake flasks is normally low [mg/L], but in fermenters, it can reach several grams per liter [g/L].63,68
Around 40% of biopharmaceutical drugs introduced on the global market today are derived from E coli gram-negative bacteria cell lines.63,64 As a result of technological advancements, E coli can now produce non-glycosylated antibody fragments and even non-glycosylated complete IgG.64,62–65 This type of cell line can produce glycosylated recombinant proteins and full-length antibodies in the future.63,69
Because of the inherent fast growth rate of Escherichia coli, high-density cultures are employed to manufacture antibody fragments. Due to the overflow metabolism of glucose, stirred tank reactors are commonly employed for E. coli production due to the production of acetate, which inhibits cell growth. Instead of relying solely on carbon sources for feeding, different metabolic engineering approaches can prevent or reduce the formation of acetate. In some cases, it may be necessary to modify the natural sugar oxidation pathway and engineer enzymes that consume glucose, while in other cases, the acetate formation pathway may need to be manipulated, and the glucose uptake system must be engineered.70
A steadily growing spectrum of protein production platforms is allowing recombinant drugs to grow in the market and expand their potential. Even though mammalian cell lines are robust manufacturers, microbial cell lines, especially E. coli, can still be effective protein factories due to their versatility and low costs.70
CONCLUSION
As market demand seems to rise for mAb, especially in cancer treatment, various methods for improving production have been introduced, including utilizing diverse types of cell lines.
This review addresses the benefits and drawbacks of the four types of expression systems. The approach is anticipated to stay dominant soon as a result of ongoing advancements in the process efficiency of mammalian cell culture.
Nevertheless, recombinant protein manufacturing is conceivable by employing several human-derived cell lines. These cells have shown a great ability for producing high-quality proteins, notably in terms of posttranslational modifications and glycosylation, allowing them to achieve comparable biopharmaceutical production rates as CHO.
Plants have significant benefits over conventional manufacturing strategies for the large-scale manufacture of recombinant proteins. Despite this, there are still some challenges to overcome to improve the quality of plant-based cell lines. E. coli and yeast strains are also discussed in this review as potent protein factories because of their versatility and cost-effectiveness. Nevertheless, because of the large range of vectors and host strains accessible in these expression systems, there is a need to optimize protein expression and secretion based on the product of interest.
In conclusion, it is critical to evaluate cell line product quality. Additionally, rather than a single host, a decent variety of diverse hosts is the most viable technique for the future, and each host system must be examined individually for each protein. It will be exciting to observe which sorts of cell lines will play an increasingly crucial role in recombinant protein manufacturing as demand for recombinant proteins develops, particularly for complicated proteins.71
References
- James K, Bell GT. Human monoclonal antibody production. Current status and future prospects. J Immunol Methods. 1987;100(1-2):5-40. [PubMed] | [CrossRef] | [Google Scholar]
- Cui Y, Cui P, Chen B, Li S, Guan H.. Monoclonal antibodies: formulations of marketed products and recent advances in novel delivery system. Drug Dev Ind Pharm. 2017;43(4):519-30. [PubMed] | [CrossRef] | [Google Scholar]
- Talotta R, Rucci F, Canti G, Scaglione F.. Pros and cons of the immunogenicity of monoclonal antibodies in cancer treatment: a lesson from autoimmune diseases. Immunotherapy. 2019;11(3):241-54. [PubMed] | [CrossRef] | [Google Scholar]
- Rita Costa A, Elisa Rodrigues M, Henriques M, Azeredo J, Oliveira R. Guidelines to cell engineering for monoclonal antibody production. Eur J Pharm Biopharm. 2010;74(2):127-38. [PubMed] | [CrossRef] | [Google Scholar]
- Lu RM Hwang YC, Liu IJ, Lee CC, Tsai HZ, Li HJ. Development of therapeutic antibodies for the treatment of diseases. J Biomed Sci.. 2020;27(1):1 [PubMed] | [CrossRef] | [Google Scholar]
- Barnard GC, Kull AR, Sharkey NS, Shaikh SS, Rittenhour AM, Burnina I, et al. High-throughput screening and selection of yeast cell lines expressing monoclonal antibodies. J Ind Microbiol Biotechnol. 2010;37(9):961-71. [PubMed] | [CrossRef] | [Google Scholar]
- Hussain H, Patel T, Ozanne AMS, Vito D, Ellis M, Hinchliffe M, et al. A comparative analysis of recombinant Fab and full-length antibody production in Chinese hamster ovary cells. Biotechnol Bioeng. 2021;118(12):4815-28. [PubMed] | [CrossRef] | [Google Scholar]
- Yang O, Prabhu S, Ierapetritou M. Comparison between batch and continuous monoclonal antibody production and economic analysis. Ind Eng Chem Res. 2019;58(15):5851-63. [CrossRef] | [Google Scholar]
- Zahavi D, Weiner L.. Monoclonal antibodies in cancer therapy. Antibodies (Basel). 2020;9(3):34 [PubMed] | [CrossRef] | [Google Scholar]
- Spadiut O, Capone S, Krainer F, Glieder A, Herwig C.. Microbials for the production of monoclonal antibodies and antibody fragments. Trends Biotechnol. 2014;32(1):54-60. [PubMed] | [CrossRef] | [Google Scholar]
- Reichert JM. Marketed therapeutic antibodies compendium. mAbs. 2012;4(3):413-5. [PubMed] | [CrossRef] | [Google Scholar]
- [PubMed] | [CrossRef] | [Google Scholar]
- Tabll A, Abbas AT, El-Kafrawy S, Wahid A.. Monoclonal antibodies: principles and applications of immunodiagnosis and immunotherapy for hepatitis C virus. World J Hepatol. 2015;7(22):2369-83. [PubMed] | [CrossRef] | [Google Scholar]
- Moussavou G, Ko K, Lee JH, Choo YK. Production of monoclonal antibodies in plants for cancer immunotherapy. BioMed Res Int. 2015;2015:306164 [PubMed] | [CrossRef] | [Google Scholar]
- Li F, Vijayasankaran N, Shen AY, Kiss R, Amanullah A.. Cell culture processes for monoclonal antibody production. mAbs. 2010;2(5):466-79. [PubMed] | [CrossRef] | [Google Scholar]
- [PubMed] | [CrossRef] | [Google Scholar]
- Kelley B. A different perspective: how much innovation is really needed for monoclonal antibody production using mammalian cell technology?. Adv Biochem Eng Biotechnol. 2018;165:443-62. [PubMed] | [CrossRef] | [Google Scholar]
- Kunert R, Reinhart D.. Advances in recombinant antibody manufacturing. Appl Microbiol Biotechnol. 2016;100(8):3451-61. [PubMed] | [CrossRef] | [Google Scholar]
- Villacrés C, Tayi VS, Butler M.. Strategic feeding of NS0 and CHO cell cultures to control glycan profiles and immunogenic epitopes of monoclonal antibodies. J Biotechnol. 2021;333:49-62. [PubMed] | [CrossRef] | [Google Scholar]
- Yu DY, Lee SY, Lee GM. Glutamine synthetase gene knockout-human embryonic kidney 293E cells for stable production of monoclonal antibodies. Biotechnol Bioeng. 2018;115(5):1367-72. [PubMed] | [CrossRef] | [Google Scholar]
- Butler M, Meneses-Acosta A.. Recent advances in technology supporting biopharmaceutical production from mammalian cells. Appl Microbiol Biotechnol. 2012;96(4):885-94. [PubMed] | [CrossRef] | [Google Scholar]
- Ho SCL, Tong YW, Yang Y.. Generation of monoclonal antibody-producing mammalian cell lines. Pharm Bioprocess. 2013;1(1):71-87. [CrossRef] | [Google Scholar]
- Dhara VG, Naik HM, Majewska NI, Betenbaugh MJ. Recombinant antibody production in CHO and NS0 cells: differences and similarities. BioDrugs. 2018;32(6):571-84. [PubMed] | [CrossRef] | [Google Scholar]
- Madabhushi SR, Podtelezhnikov AA, Murgolo N, Xu S, Lin H.. Understanding the effect of increased cell specific productivity on galactosylation of monoclonal antibodies produced using Chinese hamster ovary cells. J Biotechnol. 2021;329:92-103. [PubMed] | [CrossRef] | [Google Scholar]
- Jossé L, Zhang L, Smales CM. Application of microRNA targeted 3′UTRs to repress DHFR selection marker expression for development of recombinant antibody expressing CHO cell pools. Biotechnol J.. 2018;13(10):e1800129 [PubMed] | [CrossRef] | [Google Scholar]
- Chusainow J, Yang YS, Yeo JH, Toh PC, Asvadi P, Wong NS, et al. A study of monoclonal antibody-producing CHO cell lines: what makes a stable high producer?. Biotechnol Bioeng. 2009;102(4):1182-96. [PubMed] | [CrossRef] | [Google Scholar]
- van Berkel PH, Gerritsen J, Perdok G, Valbjørn J, Vink T, van de Winkel JG, et al. N-linked glycosylation is an important parameter for optimal selection of cell lines producing biopharmaceutical human IgG. Biotechnol Prog. 2009;25(1):244-51. [PubMed] | [CrossRef] | [Google Scholar]
- Chung CH, Mirakhur B, Chan E, Le QT, Berlin J, Morse M, et al. Cetuximab-induced anaphylaxis and IgE specific for galactose-alpha-1,3-galactose. N Engl J Med.. 2008;358(11):1109-17. [PubMed] | [CrossRef] | [Google Scholar]
- Hossler P, Khattak SF, Li ZJ. Optimal and consistent protein glycosylation in mammalian cell culture. Glycobiology. 2009;19(9):936-49. [PubMed] | [CrossRef] | [Google Scholar]
- Yee JC, Gerdtzen ZP, Hu WS. Comparative transcriptome analysis to unveil genes affecting recombinant protein productivity in mammalian cells. Biotechnol Bioeng. 2009;102(1):246-63. [PubMed] | [CrossRef] | [Google Scholar]
- Jimenez Del Val I, Fan Y, Weilguny D.. Dynamics of immature mAb glycoform secretion during CHO cell culture: an integrated modeling framework. Biotechnol J. 2016;11(5):610-23. [PubMed] | [CrossRef] | [Google Scholar]
- Chauhan G, Schmelzer AE. A novel cholesterol/lipid delivery system for murine myeloma cell lines. Biotechnol Prog. 2017;33(3):795-803. [PubMed] | [CrossRef] | [Google Scholar]
- Birch JR, Racher AJ. Antibody production. Adv Drug Deliv Rev. 2006;58(5-6):671-85. [PubMed] | [CrossRef] | [Google Scholar]
- Barnes LM, Bentley CM, Dickson AJ. Advances in animal cell recombinant protein production: GS-NS0 expression system. Cytotechnology. 2000;32(2):109-23. [PubMed] | [CrossRef] | [Google Scholar]
- Lalonde ME, Durocher Y.. Therapeutic glycoprotein production in mammalian cells. J Biotechnol. 2017;251:128-40. [PubMed] | [CrossRef] | [Google Scholar]
- Lindl T.. Development of human monoclonal antibodies: a review. Cytotechnology. 1996;21(3):183-93. [PubMed] | [CrossRef] | [Google Scholar]
- Dumont J, Euwart D, Mei B, Estes S, Kshirsagar R.. Human cell lines for biopharmaceutical manufacturing: history, status, and future perspectives. Crit Rev Biotechnol. 2016;36(6):1110-22. [PubMed] | [CrossRef] | [Google Scholar]
- Abaandou L, Quan D, Shiloach J.. Affecting HEK293 cell growth and production performance by modifying the expression of specific genes. Cells. 2021;10(7) [PubMed] | [CrossRef] | [Google Scholar]
- Beck M.. Agalsidase alfa for the treatment of Fabry disease: new data on clinical efficacy and safety. Expert Opin Biol Ther. 2009;9(2):255-61. [PubMed] | [CrossRef] | [Google Scholar]
- Loignon M, Perret S, Kelly J, Boulais D, Cass B, Bisson L, et al. Stable high volumetric production of glycosylated human recombinant IFNalpha2b in HEK293 cells. BMC Biotechnol. 2008;8:65 [PubMed] | [CrossRef] | [Google Scholar]
- Pulix M, Lukashchuk V, Smith DC, Dickson AJ. Molecular characterization of HEK293 cells as emerging versatile cell factories. Curr Opin Biotechnol. 2021;71:18-24. [PubMed] | [CrossRef] | [Google Scholar]
- Swiech K, Picanço-Castro V, Covas DT. Human cells: new platform for recombinant therapeutic protein production. Protein Expr Purif. 2012;84(1):147-53. [PubMed] | [CrossRef] | [Google Scholar]
- Dangi AK, Sinha R, Dwivedi S, Gupta SK, Shukla P.. Cell line techniques and gene editing tools for antibody production: a review. Front Pharmacol.. 2018;9:630 [PubMed] | [CrossRef] | [Google Scholar]
- Fischer R, Vasilev N, Twyman RM, Schillberg S.. High-value products from plants: the challenges of process optimization. Curr Opin Biotechnol. 2015;32:156-62. [PubMed] | [CrossRef] | [Google Scholar]
- Sack M, Rademacher T, Spiegel H, Boes A, Hellwig S, Drossard J, et al. From gene to harvest: insights into upstream process development for the GMP production of a monoclonal antibody in transgenic tobacco plants. Plant Biotechnol J. 2015;13(8):1094-105. [PubMed] | [CrossRef] | [Google Scholar]
- Xu J, Ge X, Dolan MC. Towards high-yield production of pharmaceutical proteins with plant cell suspension cultures. Biotechnol Adv. 2011;29(3):278-99. [PubMed] | [CrossRef] | [Google Scholar]
- Lomonossoff GP, D’Aoust MA. Plant-produced biopharmaceuticals: A case of technical developments driving clinical deployment. Science. 2016;353(6305):1237-40. [PubMed] | [CrossRef] | [Google Scholar]
- So Y, Lee K-J, Kim D-S, Lee J-H, Oh D-B, Hwang K-A, et al. Glycomodification and characterization of anti-colorectal cancer immunotherapeutic monoclonal antibodies in transgenic tobacco. Plant Cell Tiss Organ Cult. 2013;113(1):41-9. [CrossRef] | [Google Scholar]
- Donini M, Marusic C.. Current state-of-the-art in plant-based antibody production systems. Biotechnol Lett. 2019;41(3):335-46. [PubMed] | [CrossRef] | [Google Scholar]
- Larrick JW, Yu L, Chen J, Jaiswal S, Wycoff K.. Production of antibodies in transgenic plants. Res Immunol. 1998;149:6 [CrossRef] | [Google Scholar]
- Fischer R, Stoger E, Schillberg S, Christou P, Twyman RM. Plant-based production of biopharmaceuticals. Curr Opin Plant Biol. 2004;7(2):152-8. [PubMed] | [CrossRef] | [Google Scholar]
- Kapusta J, Modelska A, Figlerowicz M, Pniewski T, Letellier M, Lisowa O, et al. A plant-derived edible vaccine against hepatitis B virus. FASEB J.. 1999;13(13):1796-9. [PubMed] | [CrossRef] | [Google Scholar]
- Gasser B, Mattanovich D.. Antibody production with yeasts and filamentous fungi: on the road to large scale?. Biotechnol Lett.. 2007;29(2):201-12. [PubMed] | [CrossRef] | [Google Scholar]
- Mason HS, Lam DM, Arntzen CJ. Expression of hepatitis B surface antigen in transgenic plants. Proc Natl Acad Sci U S A.. 1992;89(24):11745-9. [PubMed] | [CrossRef] | [Google Scholar]
- Ma JK, Hiatt A, Hein M, Vine ND, Wang F, Stabila P, et al. Generation and assembly of secretory antibodies in plants. Science. 1995;268(5211):716-9. [PubMed] | [CrossRef] | [Google Scholar]
- Haq TA, Mason HS, Clements JD, Arntzen CJ. Oral immunization with a recombinant bacterial antigen produced in transgenic plants. Science. 1995;268(5211):714-6. [PubMed] | [CrossRef] | [Google Scholar]
- Lee YJ, Jeong KJ. Challenges to production of antibodies in bacteria and yeast. J Biosci Bioeng. 2015;120(5):483-90. [PubMed] | [CrossRef] | [Google Scholar]
- [PubMed] | [CrossRef] | [Google Scholar]
- Baghban R, Farajnia S, Rajabibazl M, Ghasemi Y, Mafi A, Hoseinpoor R, et al. Yeast expression systems: overview and recent advances. Mol Biotechnol.. 2019;61(5):365-84. [PubMed] | [CrossRef] | [Google Scholar]
- [PubMed] | [CrossRef] | [Google Scholar]
- Terpe K.. Overview of bacterial expression systems for heterologous protein production: from molecular and biochemical fundamentals to commercial systems. Appl Microbiol Biotechnol. 2006;72(2):211-22. [PubMed] | [CrossRef] | [Google Scholar]
- [PubMed] | [CrossRef] | [Google Scholar]
- Gupta SK, Shukla P.. Microbial platform technology for recombinant antibody fragment production: a review. Crit Rev Microbiol. 2017;43(1):31-42. [PubMed] | [CrossRef] | [Google Scholar]
- Walsh G.. Biopharmaceutical benchmarks 2010. Nat Biotechnol. 2010;28(9):917-24. [PubMed] | [CrossRef] | [Google Scholar]
- Rosano GL, Ceccarelli EA. Recombinant protein expression in : advances and challenges. Front Microbiol. 2014;5:172 [PubMed] | [CrossRef] | [Google Scholar]
- Choi JH, Lee SY. Secretory and extracellular production of recombinant proteins using . Appl Microbiol Biotechnol. 2004;64(5):625-35. [PubMed] | [CrossRef] | [Google Scholar]
- Kipriyanov SM, Moldenhauer G, Little M.. High-level production of soluble single chain antibodies in small-scale cultures. J Immunol Methods. 1997;200(1-2):69-77. [PubMed] | [CrossRef] | [Google Scholar]
- Makrides SC. Strategies for achieving high-level expression of genes in . Microbiol Rev.. 1996;60(3):512-38. [PubMed] | [CrossRef] | [Google Scholar]
- Lizak C, Fan YY, Weber TC, Aebi M.. N-Linked glycosylation of antibody fragments in . Bioconjug Chem. 2011;22(3):488-96. [PubMed] | [CrossRef] | [Google Scholar]
- Sanchez-Garcia L, Martín L, Mangues R, Ferrer-Miralles N, Vázquez E, Villaverde A., et al. Recombinant pharmaceuticals from microbial cells: a 2015 update. Microb Cell Factories. 2016;15:33 [PubMed] | [CrossRef] | [Google Scholar]
- Javanmard Sh. An overview of the various appropriate types of cell lines for the production of monoclonal antibodies. Authorea [preprint]. 2022 [CrossRef] | [Google Scholar]