ABSTRACT
Background
Herbal medicines have been valued for centuries as potent treatments for various conditions, including inflammatory and microbial infections. Urochloa distachya (Signal grass), an annual grass belonging to the Poaceae family, is characterized by lance-shaped leaves and 2-3 racemes. This plant is native to tropical regions of Asia and Africa but has also spread to other regions. It thrives in hot, humid climates, particularly during monsoon seasons. Materials and methods: The anti-inflammatory activity was evaluated using the carrageenan-induced paw edema model in experimental animals. Paw edema was induced by injecting carrageenan into the hind paw, and the extent of inflammation was measured at specific time intervals. The antimicrobial activity was assessed using the disk diffusion method. The zone of inhibition around the discs was measured to determine antimicrobial efficacy.
Results
GC-MS analysis of the essential oil of Urochloa distachya identified eight phytoconstituents, Phytol (59.91%) is identified as the highest peak compound. The anti-inflammatory properties of the plant were evaluated using a carrageenan-induced paw edema model in rats. At a dose of 400 mg/kg, the essential oil significantly (p<0.05) reduced paw edema, achieving a notable inhibition rate of 56.5% at the 4th hr. The antibacterial activity was evaluated against S. typhi and S. aureus. Molecular docking study was performed using protein COX-2, IL-1β, NF-κB, Phospholipase and TNF-α. β-Carotene showed the highest docking scores of -9.0, -7.8, -7.6, -7.3 and -7.7 against COX-2, IL-1β, NF-κB, Phospholipase and TNF-α, respectively. Similarly, docking scores of -7.7, -7.8 and -7.7 were observed against Dihydropteroate synthase, Transpeptidase and Type I dehydroquinase.
Conclusion
Urochloa distachya exhibits substantial potential as a natural source of essential oils with potent anti-inflammatory and antimicrobial properties, underscoring its potential for future therapeutic applications.
INTRODUCTION
Inflammation is an immune system response to a variety of harmful stimuli, including burns, allergies, mechanical injuries and other toxic stimuli (Thitinarongwateet al., 2022). It is an immune response that is thought to eliminate the major cause of cellular injury, destroy dead tissue and cells and stimulate healing. Although inflammation is beneficial if acute and contained, it will cause tissue injury and thus contribute to the pathogenesis of some chronic diseases if it is out of balance (Medzhitov, 2008). Inflammation can be categorized as acute or chronic. Acute is an instantaneous reaction to injury, generally persisting for up to 2 hr, but chronic inflammation is an extended response linked to long-term medical issues (Anyasoret al., 2019). Chronic inflammation is a primary factor in the pathogenesis of various ailments, which include Rheumatoid Arthritis (RA) and lupus, diabetes, obesity and atherosclerosis. Moreover, it has been associated with the onset and advancement of cancer, especially in tissue exhibiting persistent inflammatory reactions (Medzhitov, 2010). Anti-inflammatory drugs play a vital role in managing and treating conditions associated with excessive or chronic inflammation. These substances function by preventing tissue damage, reducing the synthesis of pro-inflammatory mediators and regulating the inflammatory response (Azabet al., 2016). Anti-inflammatory agents are categorized into Steroidal Anti-Inflammatory Drugs (SAIDs), which includes corticosteroids and Non-Steroidal Anti-Inflammatory Drugs (NSAIDs), like aspirin and ibuprofen. These agents target key pathways, including Cyclooxygenase (COX) enzyme and Nuclear Factor-kappa B (NF-κB), to mitigate inflammation. Recent advancements have underscored the efficacy of biologics, particularly monoclonal antibodies and natural compounds like curcumin, in delivering targeted anti-inflammatory therapy (Charles-Messanceet al., 2020).
Microorganisms, such as bacteria, fungi, viruses and archaea, are found everywhere and are essential for sustaining ecological balance and supporting human health. Microbial studies have progressed notably, emphasizing their roles in biogeochemical cycles, industrial processes and human physiology (Berget al., 2020). The human microbiome has been demonstrated to impact metabolic processes, immune system development and susceptibility to diseases. Concurrently, harmful microbes contribute to a range of infectious diseases, highlighting the urgent need for the formulation of effective strategies to address microbial threats (Marchesi and Ravel, 2015). The global healthcare system faces a critical challenge in the urgent demand for new, effective and affordable drugs to address microbial infections. Antimicrobial Resistance (AMR) has significantly reduced the efficacy of traditional treatments, leading to higher mortality rates and increased healthcare expenditure (Murray et al., 2022). Public health is increasingly threatened by pathogens such as multidrug-resistant Escherichia coli, Methicillin-Resistant Staphylococcus Aureus (MRSA) and extensively drug-resistant Mycobacterium tuberculosis. Additionally, emerging infectious diseases, including those caused by SARS-CoV-2, further exacerbate the strain on existing drug development efforts (Tacconelliet al., 2018). Despite this pressing need, progress in discovering and developing new antimicrobial agents has slowed due to economic and scientific hurdles. To tackle this crisis, innovative strategies such as natural product screening, synthetic biology and antimicrobial peptides, alongside global cooperative efforts, are crucial for ensuring the availability of effective and affordable treatments (WHO, 2019).
Herbal drugs have been utilized for centuries as effective remedies for various ailments, including inflammatory and microbial infections. These natural products, derived from plants, contain bioactive compounds such as alkaloids (Soutoet al., 2011; Yanet al., 2021), flavonoids (B. Singh and Sharma, 2015), tannins (Kováč et al., 2023) and terpenoids (Yanget al., 2020) that exhibit significant anti-inflammatory and antimicrobial properties. Herbal extracts, such as those from Curcuma longa (turmeric) (Ifyet al., 2021), Azadirachta indica (neem) (Kauret al., 2022) and Eucalyptus globulus (eucalyptus) (Salvatoriet al., 2023), have demonstrated efficacy in modulating inflammatory pathways and inhibiting microbial growth.
Urochloa distachya (Signal grass) is an annual Poaceae grass with lance-shaped leaves and 2-3 racemes, it originates from tropical Asia, Africa and other regions. It thrives well in hot and humid weather, especially during the monsoon season (Dashet al., 2023). Previous studies on the petroleum ether extract of U. distachya have revealed the presence of key phytoconstituents, including Phytol, γ-Sitosterol and α-Amyrin. These bioactive compounds are widely recognized for their potent anti-inflammatory and antimicrobial properties (Dashet al., 2023; Islamet al., 2018; Loizouet al., 2010; Yadavet al., 2024; Vietet al., 2021; Okoyeet al., 2014). Another study on the methanolic leaf extract of U. distachya identified various phytoconstituents, many of which are known for their anti-inflammatory and antimicrobial activities. Notable compounds include 2-[4-Methyl-6-(2,6,6-trimethylcyclohex-1-enyl)hexa-1,3,5-trienyl]cyclohex-1-en-1-carboxaldehyde; 7-Methyl-Z-tetradecen-1-ol acetate; 2-Naphthalenol, 2,3, 4,4a,5,6,7-octahydro-1,4a-dimethyl-7-(2- hydroxy-1-methylethyl)-; Spiro[4.5]decan-7-one, 1,8-dimethyl-8,9-epoxy-4-isopropyl; 9,12,15-Octadecatrienoic acid, 2,3-bis[(trimethylsilyl)oxy]propyl ester, (Z, Z, Z); and Diisooctyl phthalate (Dashet al., 2022). This study aims to explore the anti-inflammatory properties and antimicrobial potential of the essential oil extracted from Urochloa distachya (L.).
MATERIALS AND METHODS
Chemicals and reagents
Carrageenan (Sigma Lambda, USA), Indomethacin (Micro Labs Ltd.,) CMC (Sigma-Aldrich) and Ciprofloxacin (Cipla Ltd.).
Collection of plant materials
U. distachya plants were gathered from the village Hatgaon, Odisha, India and identified by Dr. V. Ranjan (Scientist D) at BSI, Howrah, Kolkata, India, with voucher number CNH/Tech.II/2019/77. The plant materials were deposited in the pharmacognosy department for subsequent use.
Preparation of essential oil
The dried whole plant materials (1000 g) of U. distachya were coarsely grounded and subjected to hydro-distillation with a Clevenger apparatus. The obtained oil was filtered and stored in vials at 4°C until further study (Khandelwal, 2007).
Experimental animals
Eighteen Wistar albino rats (8-10 weeks old, weighing 160-170 g) were procured from M.s Chakraborty Enterprises (Reg. No. 1443/PO/Bt/s/CPCSEA, Kolkata) for anti-inflammatory study. The rats underwent a 7-day acclimatization period under controlled conditions (24 hr dark and light cycle, 22±3ºC) with free access to food and water. Cages were cleaned regularly to ensure hygiene. The study followed ethical guidelines for the care and use of laboratory animals. Using the Carrageenan-Induced Paw Edema (CIPE) model, U. distachya exhibited notable anti-inflammatory activity, confirming the model’s reliability.
Ethical statement
All experiments complied with international guidelines and received approval from the Animal Ethical Committee (1376/PO/Re/S/10/CPCSEA) under project proposal number IAEC/01/2024 for the acute toxicity and IAEC/03/2024 for the CIPE model in rats.
GC-MS analysis
GC-MS analysis utilized Agilent 5977 MSD technology, employing an HP-5 MS fused silica column (30 m×250 μM×0.25 μM) coupled to an MSD interface. Helium was used as the carrier gas at a flow rate of 1.2 mL/min. The column temperature varied between 60ºC and 325ºC at a pressure of 11.367 psi, with a total duration of 40 min. A 1 μL sample was injected and mass determination was performed at 70 eV. The quadrupole and source temperatures were established at 150ºC and 230ºC, respectively, for 10 min. The chromatogram provided a quantification of chemical concentrations in EOUD expressed as percentages (Dashet al., 2023).
Acute toxicity study
Acute oral toxicity was conducted following OECD guideline 423 (Annexure 2b) using Wistar albino rats (Anonymous, 2001). Three rats were administered a 2000mg/kg dose of EOUD and observed for behavioral changes during the first 4 hr, followed by daily observation for 14 days for any clinical issue or toxicological signs. No signs of toxicity or mortality were observed during the study. A dose of 400 mg/kg of EOUD demonstrated significant safety for anti-inflammatory activity.
Carrageenan-induced paw edema
The rats were divided into three groups (n=6) for assessment of the anti-inflammatory effect using carrageenan-induced paw edema. Each group received a 0.1 mL injection of 1% w/v carrageenan in 1% CMC into the left hind paw (M. Singhet al., 2010).
- Group I (Control): Carrageenan only,
- Group II (Positive control): Indomethacin 10 mg/kg b.w. orally+carrageenan,
- Group III (Test control): EOUD 400 mg/kg b.w. orally+carrageenan.
Paw thickness was evaluated with a vernier caliper before treatment and at 60, 120, 180 and 240 min after carrageenan injection, Anti-inflammatory effect was calculated by determining the percentage inhibition of edema relative to the control group using the following formula.
Where Tt is the thickness of the paw of the test group and T0 is the paw thickness of the control group.
Antibacterial activity
The antimicrobial activity of the extracts was assessed using the disk diffusion method against Salmonella typhi and Staphylococcus aureus. The strains were sourced from CSIR, Chandigarh, India. A freshly prepared liquid culture of the test pathogens (S. typhi and S. aureus) was evenly spread onto nutrient agar plates using a sterile swab. Sterile filter paper discs, impregnated with various concentrations of standard ciprofloxacin (0.625, 1.25, 2.5 and 5 µg/mL) and 100 µL of EOUD, were placed on the agar surface. The plates were then incubated at 37ºC for 18-24 hr. The presence of a clear zone around the disc indicated antimicrobial activity, which was measured to find out the inhibition activity, which was measured to determine the inhibition zone. All experiments were performed in triplicate and the mean+SD of the inhibition zone was calculated to evaluate the antibacterial properties of the extracts (Pandaet al., 2014).
In silico molecular docking
In silico molecular docking studies were carried out to assess the anti-inflammatory and antibacterial properties of the phytoconstituents identified from GC-MS analysis of EOUD. For anti-inflammatory activity, the 3D structure of key targets was utilized, including Cyclooxygenase-2 (COX-2, PDB ID: 5f19) (Lucidoet al., 2016), Interleukin-1 beta (IL-beta, PDB ID: 4g6m) (Blechet al., 2013), Nuclear Factor Kappa B (NF-kB, PDB ID: 1nfi) (Jacobs and Harrison, 1998), Phospholipase A2 (PDB ID: 1dcy) (Schevitzet al., 1995) and Tumor Necrosis Factor Alpha (TNF-alpha, PDB ID: 2az5) (Heet al., 2005). For antimicrobial activity, the selected target included Dihydropteroate synthase (PDB ID: 1ad4) (Hampeleet al., 1997), Transpeptidase (PDB ID: 5tw8) (Alexanderet al., 2018) and Type I Dehydroquinase (PDB ID: 4cno) (Maneiroet al., 2014). These protein targets were chosen to investigate the potential therapeutic mechanisms of the bioactive compounds. The 3D and 2D structure of compounds were taken from PubChem bearing Ids, Indomethacin (3715), Ciprofloxacin (2764), Docosanoic acid, 1,2,3-propanetriyl ester (62726), Octadecanal, 2-bromo- (537255), Phytol (5280435), Pentadecanoic acid (13849), Tristearin (11146), E-3-Pentadecen-2-ol (5363322), β Carotene (5280489). Proteins were prepared using AutoDock Tools version 1.5.7 and docking was conducted on the crystallographic structure of chain A. To identify the active site, polar hydrogens were added and Kollman charges were applied. Ligand structures were generated using Open Babel software (v. 2. 4. 1). The active regions were identified as the amino acid residue that interacts with the ligands, as indicated in the protein files. To cover the binding area of the protein a grid box was made and set to have 60x60x60 x, y and z points. The grid center was set at 20.875, 37.550 and 59.367 for COX-2; 4.168, 29.893 and -26.024 for IL-1β; -3.961, 47.607 and 13.038 for NF-KAPPA; 59.081, 32.277 and 41.396 for Phospholipase A2; -26.420, 65.920 and 41.976 for TNF-α; 34.588, 8.016 and 42.320 for Dihydropteroate synthase; 22.167, -59.861 and 38.111 for Transpeptidase; 27.984, -0.129 and 19.373 for Type I dehydroquinase. The docking analysis was performed using AutoDock Vina to calculate the binding scores, while the 3D visualization and analysis of protein-ligand interactions were performed using the Biovia Discovery Studio 2021 Client (Dash et al., 2024).
Statistical analysis
Statistical data was calculated by using one-way ANOVA, followed by Tukey’s HSD post hoc test, utilizing SPSS (version 21, IBM Corporation) and MS Excel (version 2019). Results are stated as mean±S.E.M. (standard error of the mean), with P-values below 0.05 regarded as statistically significant.
RESULTS
GC-MS analysis of EOUD
GC-MS analysis of EOUD identified eight phytoconstituents, with four major compounds, Phytol (59.91%); Octadecanal, 2-bromo- (15.82%); Docosanoic acid, 1,2,3-propanetriyl ester (6.50%) and Pentadecanoic acid (5.32%). The remaining compounds, considered minor constituents, include β Carotene (3.96%), Tristearin (3.04%); 1,3-Dipalmitin trimethylsilyl ether (2.88%); and E-3-Pentadecen-2-ol (2.58%). Notably, nearly all identified compounds possess documented anti-inflammatory and antimicrobial activity, making them pivotal in evaluating EOUD’s therapeutic value in these areas. Detailed information about each compound, including its retention time, chemical nature, molecular formula, molecular weight and associated biological activities, is presented in Table 1. Additionally, the chromatographic profiles are illustrated in Figures 1 and 2.
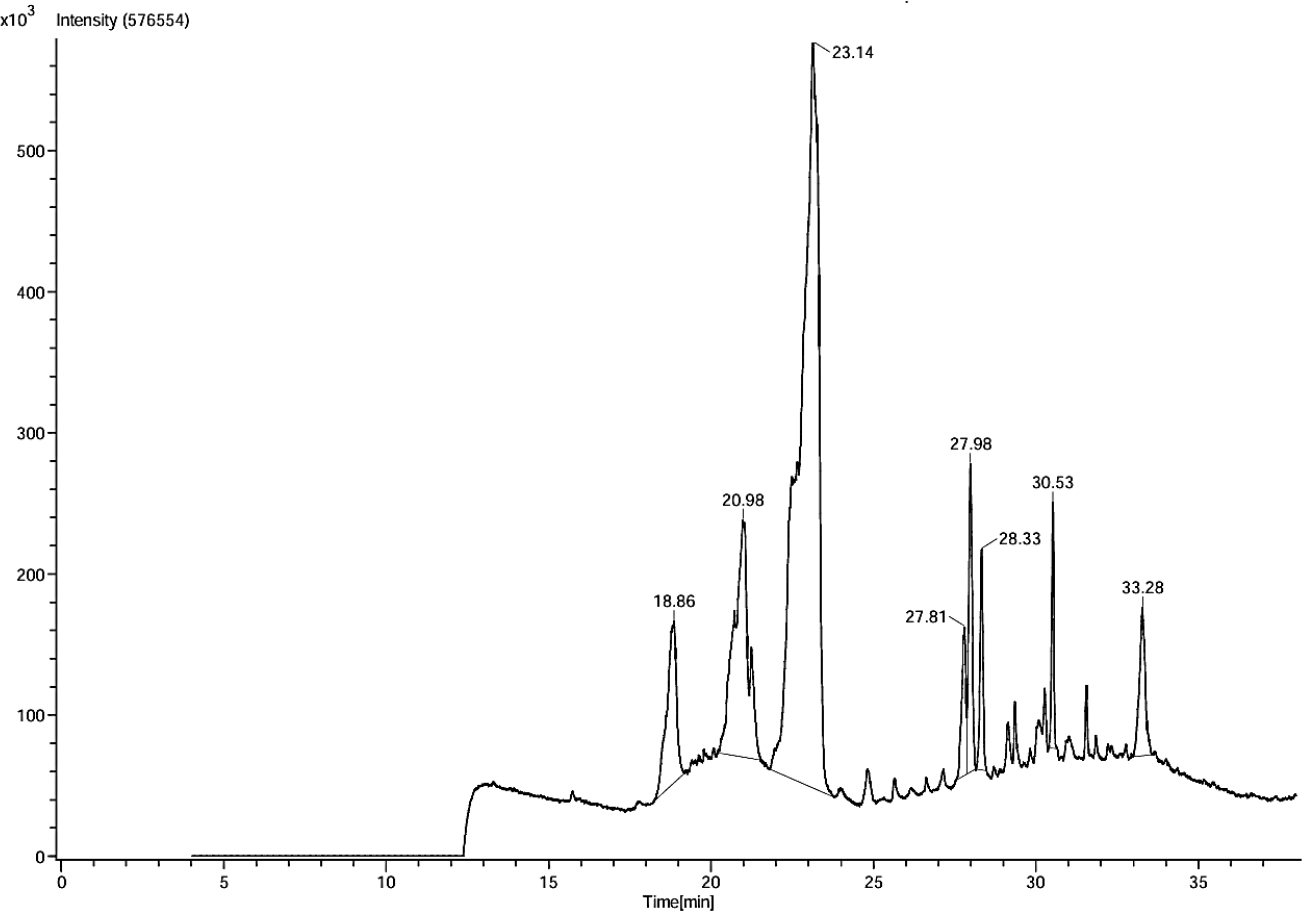
Figure 1:
GC-MS chromatogram of U. distachya essential oil.
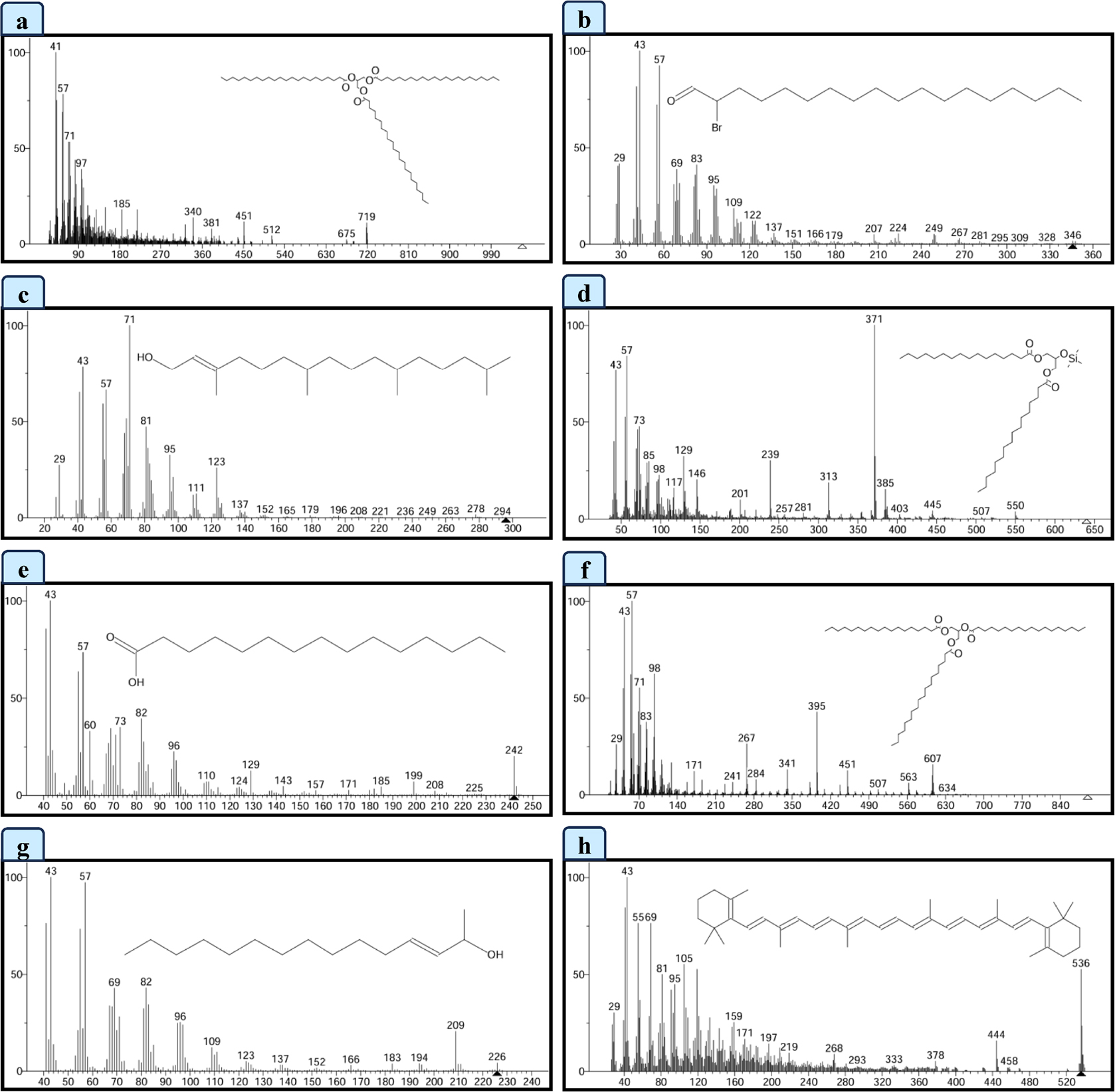
Figure 2:
GC-MS spectral data of (a) Docosanoic acid, 1, 2, 3-propanetriyl ester, (b) Octadecanal, 2-bromo-, (c) Phytol, (d) 1,3-Dipalmitin trimethylsilyl ether, (e) Pentadecanoic acid, (f) Tristearin, (g) E-3-Pentadecen-2-ol, (h) β Carotene.
Sl. No. | RT (min) | Name of the compound | Nature of compound | M. F. | M. W. | Peak area% | Activity | References |
---|---|---|---|---|---|---|---|---|
1 | 18.86 | Docosanoic acid, 1, 2, 3-propanetriyl ester | Ester | C69H134O6 | 1059.8 | 6.50 | Antimicrobial activity, Antioxidant, nematicide, hypocholesterolemic, pesticide, anti-androgenic activity, lubricant and flavoring agent. | (Geethaet al., 2013) |
2 | 20.98 | Octadecanal, 2-bromo- | Long-chain aldehyde | C18H35BrO | 347.4 | 15.82 | Antifungal, antimicrobial activity, Anti-apoptotic, anti-inflammatory activity. | (Dinesh Kumar Get al., 2018; Nisaet al., 2022) |
3 | 23.14 | Phytol | Acyclic diterpenoid alcohol | C20H40O | 296.5 | 59.91 | Anti-inflammatory, antioxidant, antianxiety, cytotoxicity, antimicrobial. | (Islamet al., 2018) |
4 | 27.81 | 1,3-Dipalmitin trimethylsilyl ether | Organic compound | C38H76O5Si | 641.1 | 2.88 | Antifungal agent. | (Ábrego-Garcíaet al., 2022) |
5 | 27.98 | Pentadecanoic acid | Odd-chain saturated fatty acid | C15H30O2 | 242.40 | 5.32 | Anti-inflammatory, antifibrotic activity. | (Venn-Watsonet al., 2020) |
6 | 28.33 | Tristearin | Saturated triglyceride | C57H110O6 | 891.5 | 3.04 | Antioxidant activity. | (Chanet al., 1996) |
7 | 30.53 | E-3-Pentadecen-2-ol | Alcohols | C15H30O | 226.40 | 2.58 | Not reported | |
8 | 33.28 | β Carotene | Carotenoid compound | C40H56 | 536.9 | 3.96 | Antioxidant, anti-inflammatory, anti-tumor activity. | (Wuet al., 2023) |
GC-MS analysis of essential oil of U. distachya.
Anti-inflammatory activity
Carrageenan-Induced Paw Edema (CIPE)
The anti-inflammatory activity of the essential oil of U. distachya was evaluated using a CIPE model in rats. The carrageenan injection caused a significant increase in paw thickness, indicating inflammation. However, treatment with EOUD at a dose of 400 mg/kg significantly (p<0.05) reduced paw edema with a notable inhibition percentage of 56.5% at the 4th hr. While the standard drug achieved an inhibition of 83.2%, the results highlight the promising anti-inflammatory effects of EOUD. The measurements from the 1st hr to the 4th hr are presented in Table 2 and effects are shown in Figure 3.
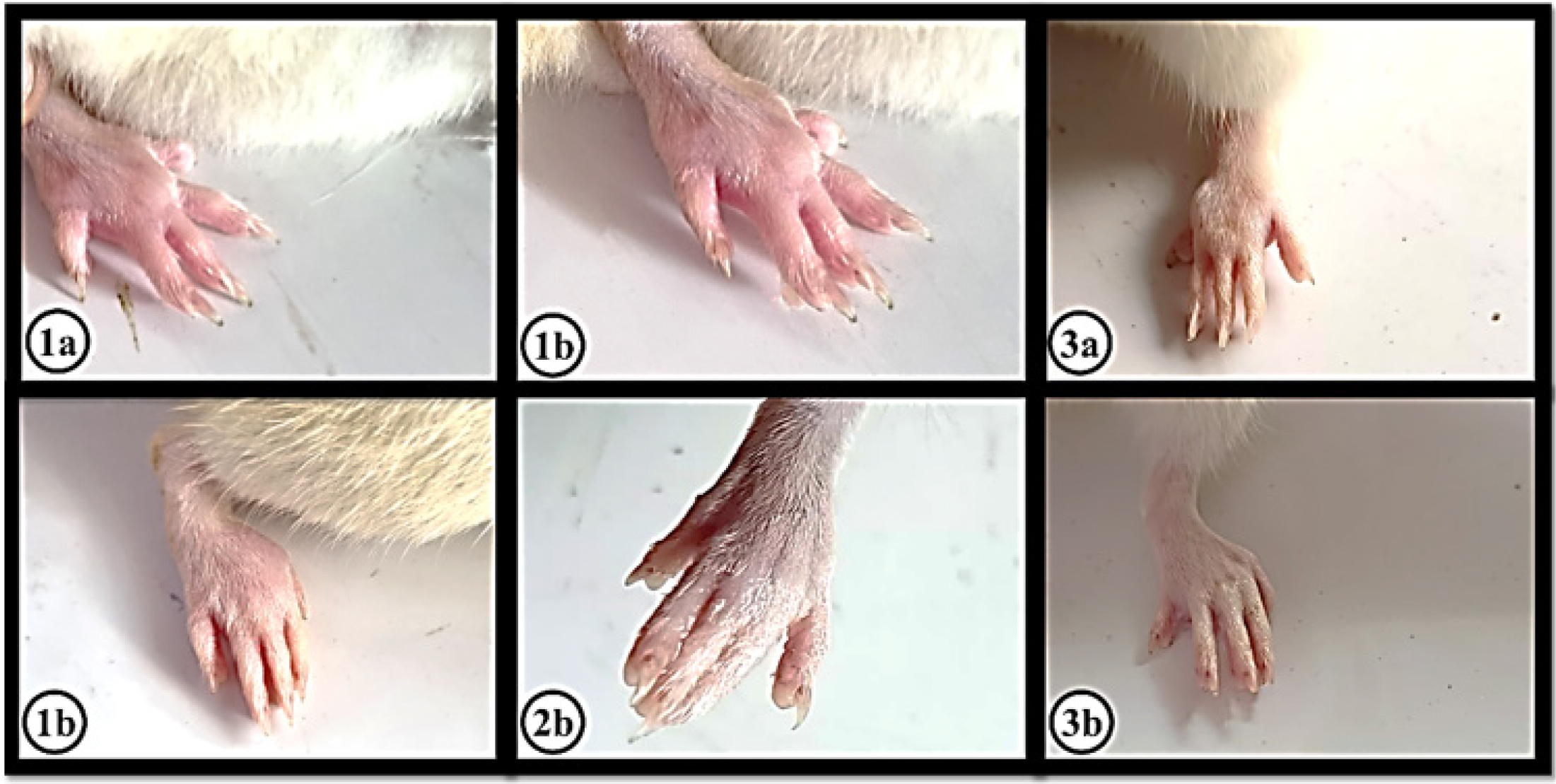
Figure 3:
The effect of carrageenan, indomethacin and EOUD on inflammation in the left hind paw of rats was measured at 1 hr and 4 hr post-treatment. (1a) and (1b) showed the effect of Carrageenan induced (0.1 mL of 1% w/v). (2a) and (2b) showed the effect of Indomethacin 10 mg/kg b.w. (3a) and (3b) showed the effect of Carrageenan induced (0.1 mL of 1% w/v)+EOUD 400 mg/kg b.w.
Group | Treatment | Change in paw thickness (mm)±SD (% inhibition) | |||
---|---|---|---|---|---|
1st hr | 2nd hr | 3rd hr | 4th hr | ||
Group I: Carrageenan Control | Carrageenan (0.1 mL of 1% w/v) | 3.32±0.02c | 4.19±0.06c | 3.21±0.04 c | 2.97±0.01c |
Group II: Positive Control | Carrageenan (0.1 mL of 1% w/v)+Indomethacin 10 mg/kg b.w. | 2.61±0.02a*21.39% | 3.11±0.02a*69.17% | 2.54±0.03b*73.52% | 2.22±0.03a*83.2% |
Group III: EOUD | Carrageenan (0.1 mL of 1% w/v)+ EOUD 400 mg/kg b.w. | 2.71±0.02b*49.60% | 3.21±0.02b*57.68% | 2.50±0.23a*55.14% | 2.45±0.19b*56.5% |
In vivo anti-inflammatory activity of EOUD.
Antimicrobial activity
The antibacterial activity of the essential oil of U. distachya was evaluated against S. typhi and S. aureus. For this study, paper disks were soaked in 100 µL of EOUD and placed on the agar surface inoculated with the bacterial strains. The results showed that the EOUD produced a zone of inhibition measuring 25.80±0.20 mL against S. typhi and 23.87±0.20 mM against S. aureus. In comparison, the standard drug ciprofloxacin, at a dose of 5 µg/mL, exhibited a zone of inhibition of 20.07±0.21 mM for S. typhi and 21.97±0.20 mM for S. aureus (Table 3 and Figure 4).
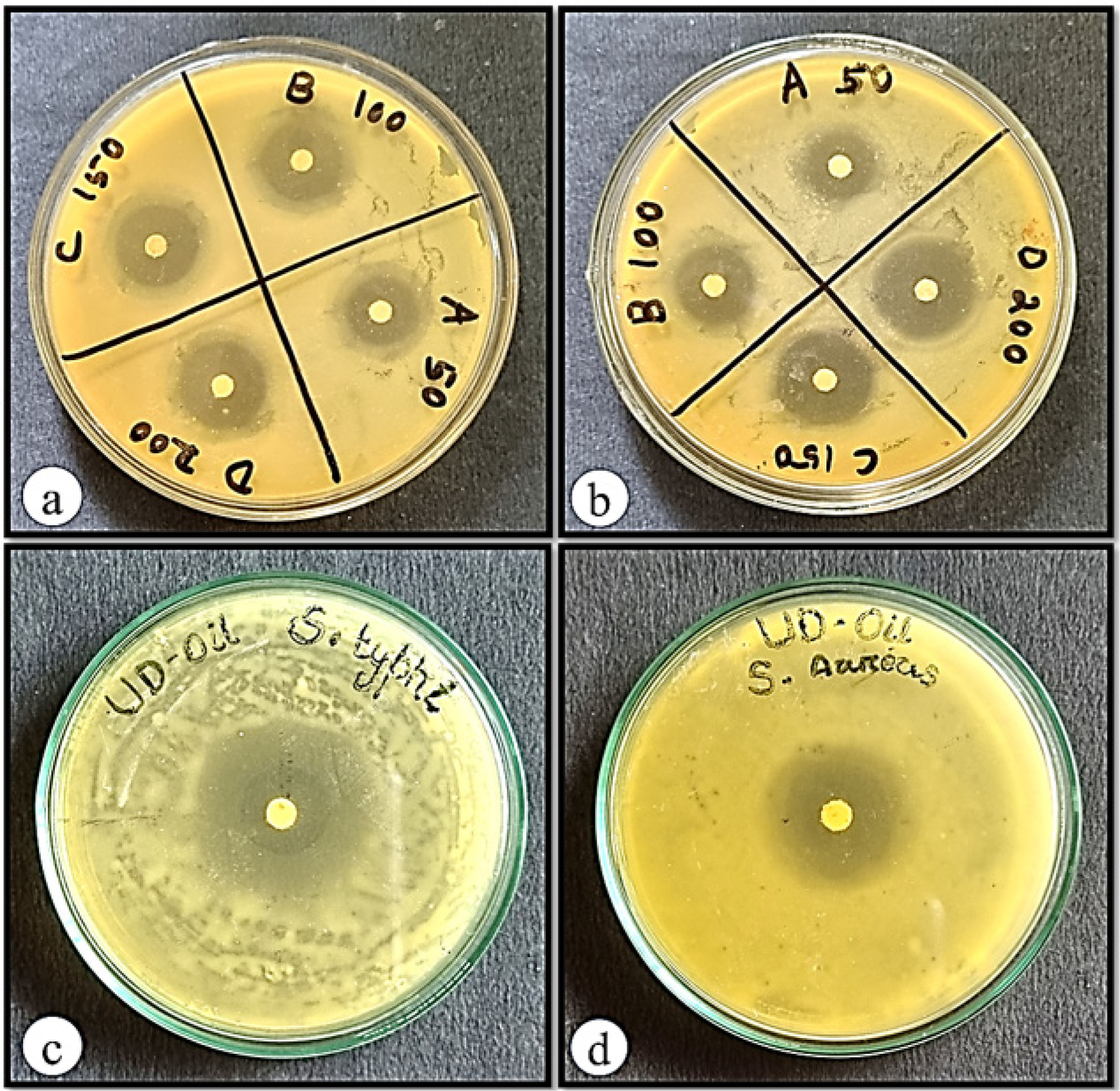
Figure 4:
Antibacterial activity of (a) ciprofloxacin against S. typhi, (b) ciprofloxacin against S. aureus, (c) essential oil of U. distachya against S. typhi and (d) essential oil of U. distachya against S. aureus.
Treatment | Dose | Zone of inhibition (mm) | |
---|---|---|---|
S. typhi (Gram negative) | S. aureus (Gram positive) | ||
Ciprofloxacin | 0.625 µg/mL | 12.73±0.25 | 12.00±0.20 |
1.25 µg/mL | 14.97±0.15 | 14.03±0.20 | |
2.5 µg/mL | 16.83±0.15 | 18.00±0.20 | |
5 µg/mL | 20.07±0.21 | 21.97±0.20 | |
EOUD | 100 µL | 25.80±0.20 | 23.87±0.20 |
Antibacterial activity of EOUD.
Molecular docking
Molecular docking analyses were carried out using protein COX-2, IL-1β, NF-κB, Phospholipase and TNF-α. Compounds identified through GC-MS analysis were evaluated for their docking scores, which ranged from -4.5 to -9 for COX-2, -3.6 to -7.8 for IL-1β, -3.4 to -7.6 for NF-κB, -5.4 to -7.3 for phospholipase A2 and -3.4 to -7.7 for TNF-α. Among the revealed phytoconstituents, β Carotene exhibited the highest docking scores of -9.0, -7.8, -7.6, -7.3 and -7.7 against COX-2, IL-1β, NF-κB, Phospholipase and TNF-α respectively (Table 4). The ranges of docking score of the compounds form the protein, Dihydropteroate synthase -3.5 to -7.7, Transpeptidase -3.4 to -7.8 and Type I dehydroquinase -3.8 to -7.7 (Table 6). The details study of hydrogen and hydrophobic interactions is presented in Tables 5 and 7 and the binding affinities are illustrated in Figures 5–12.
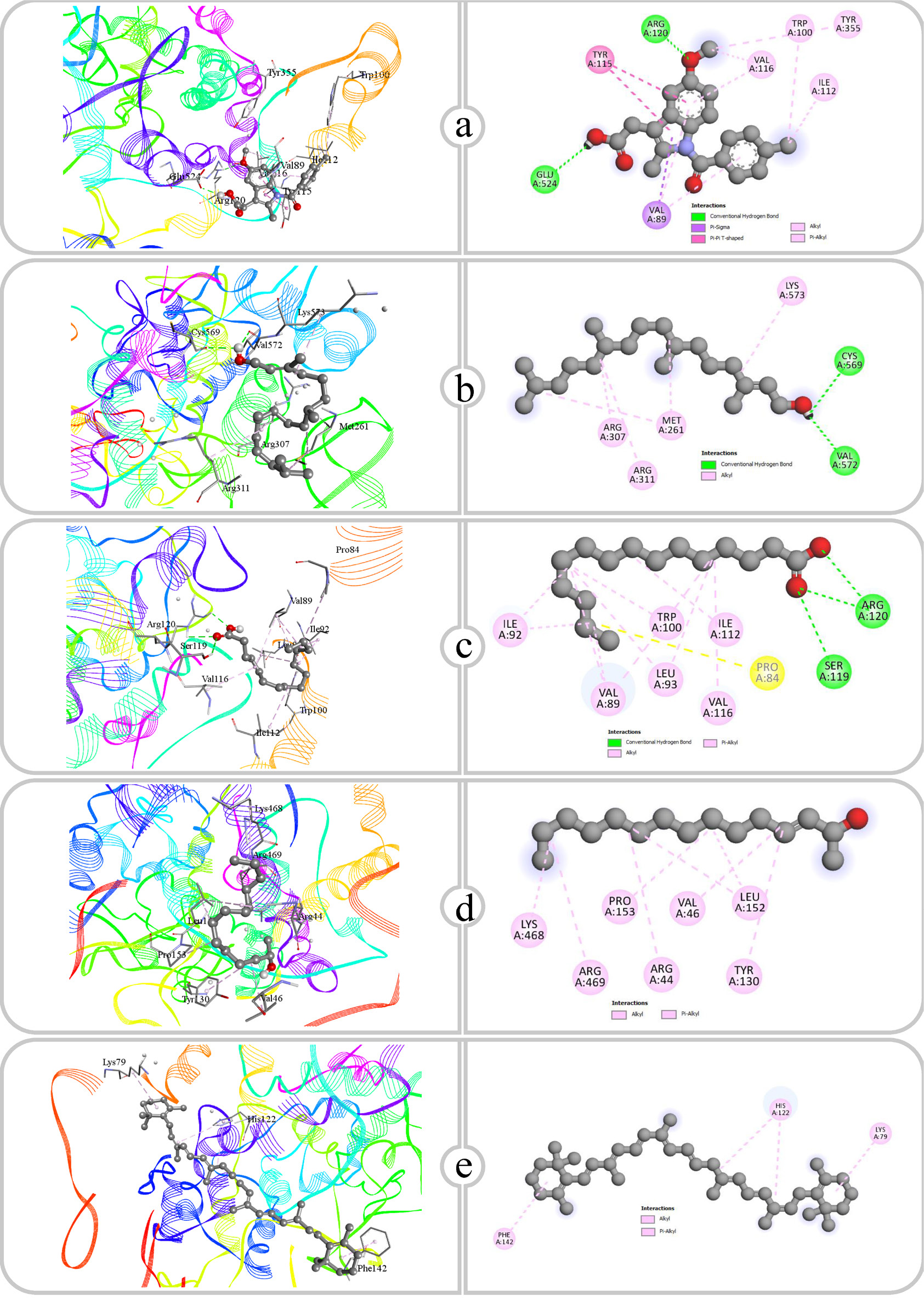
Figure 5:
Binding affinity and molecular interaction of (a) Indomethacin, (b) Phytol, (c) Pentadecanoic acid, (d) E-3-Pentadecen-2-ol and (e) β Carotene against COX-2 (5f19).
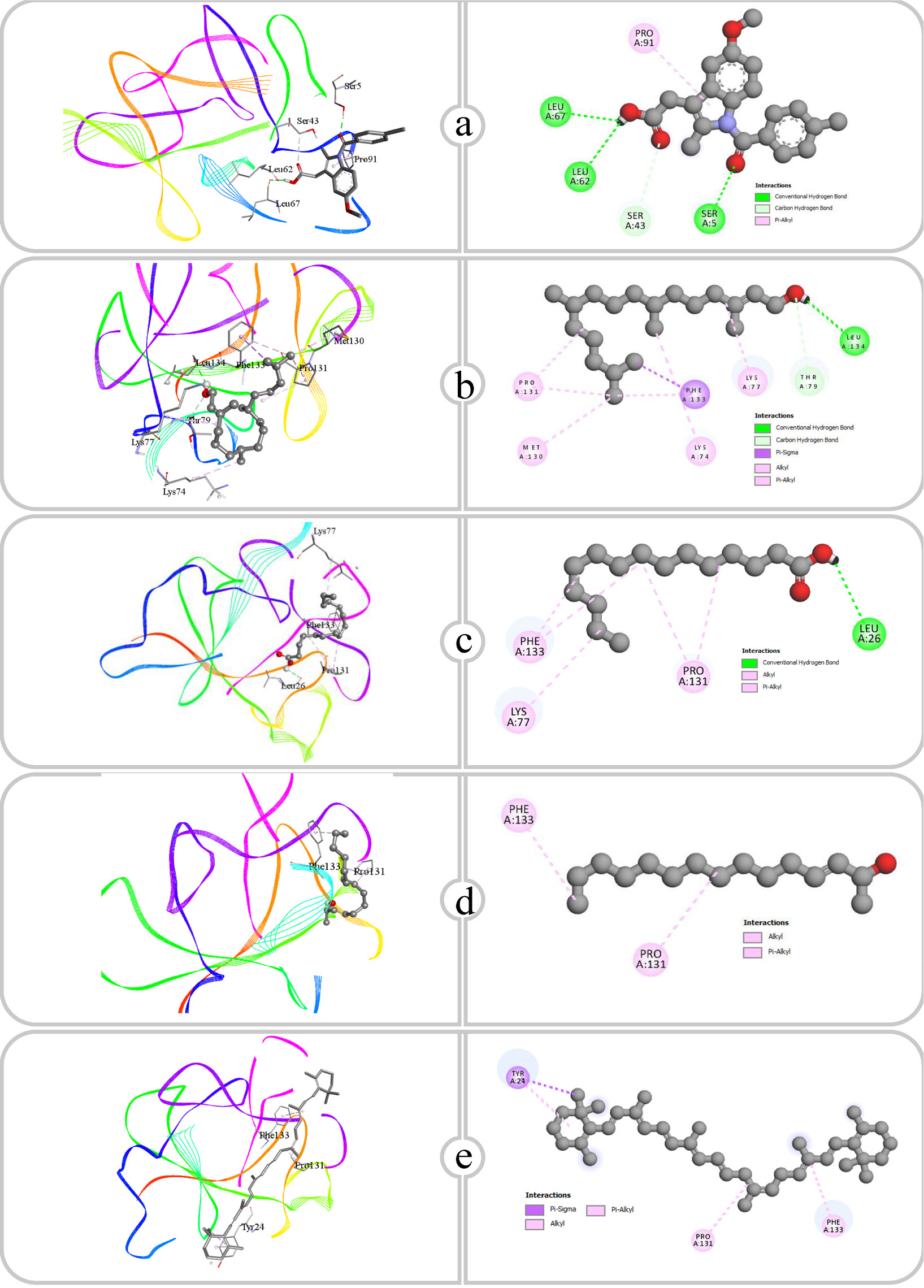
Figure 6:
Binding affinity and molecular interaction of (a) Indomethacin, (b) Phytol, (c) Pentadecanoic acid, (d) E-3-Pentadecen-2-ol and (e) β Carotene against IL-1β (4g6m).
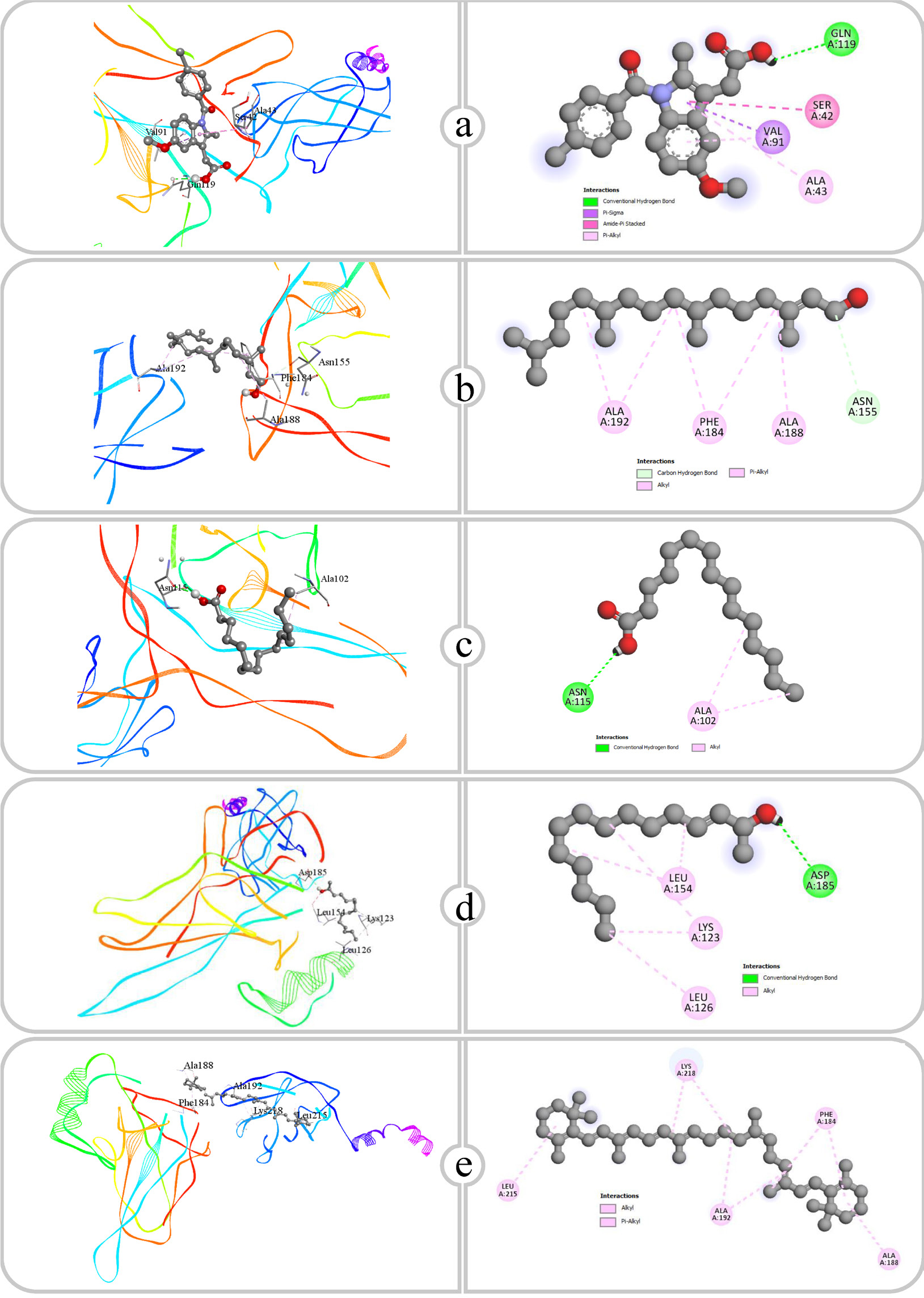
Figure 7:
Binding affinity and molecular interaction of (a) Indomethacin, (b) Phytol, (c) Pentadecanoic acid, (d) E-3-Pentadecen-2-ol and (e) β Carotene against NF-KAPPA (1nfi).
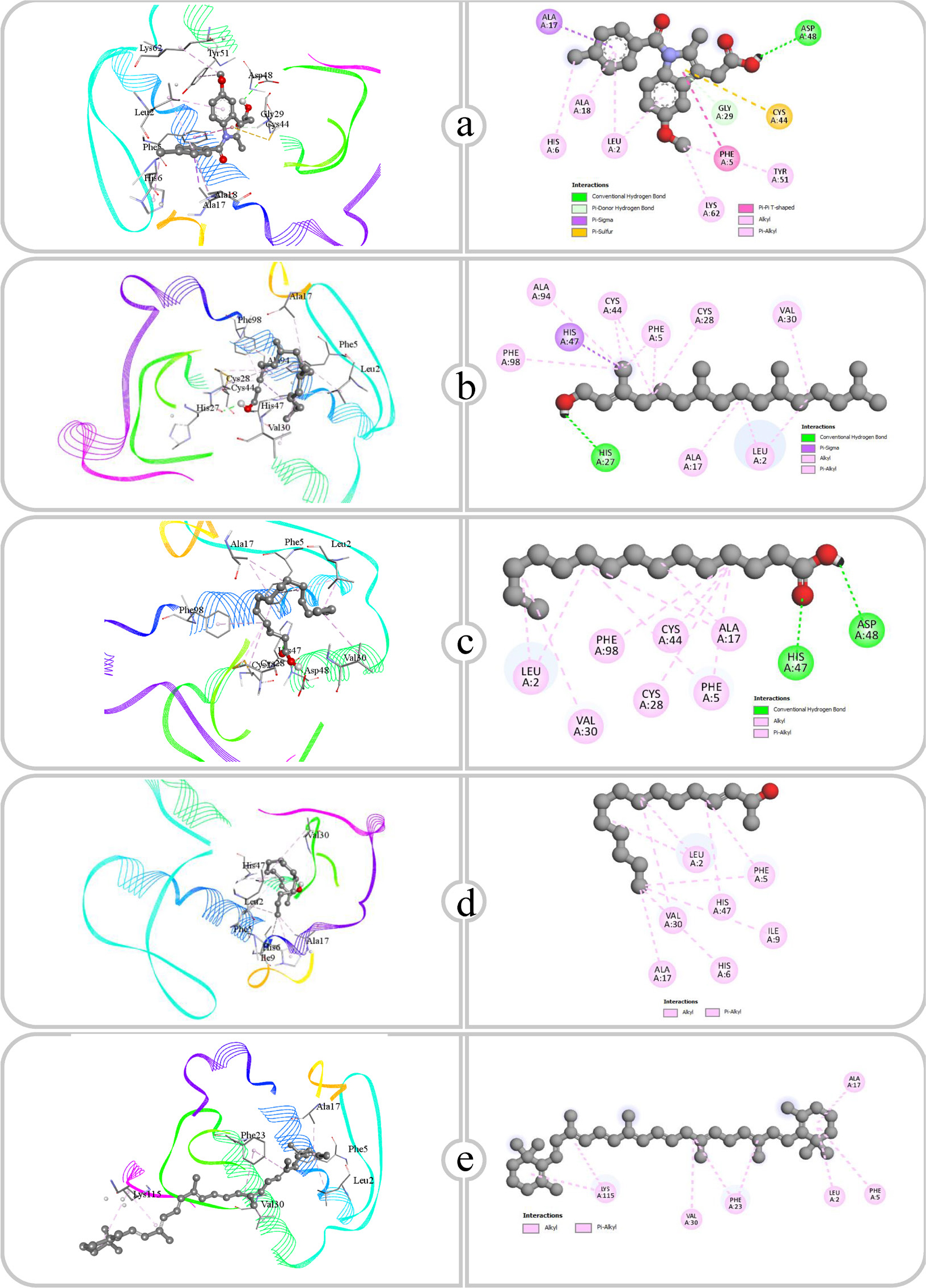
Figure 8:
Binding affinity and molecular interaction of (a) Indomethacin, (b) Phytol, (c) Pentadecanoic acid, (d) E-3-Pentadecen-2-ol and (e) β Carotene against Phospholipase A2 (1dcy).
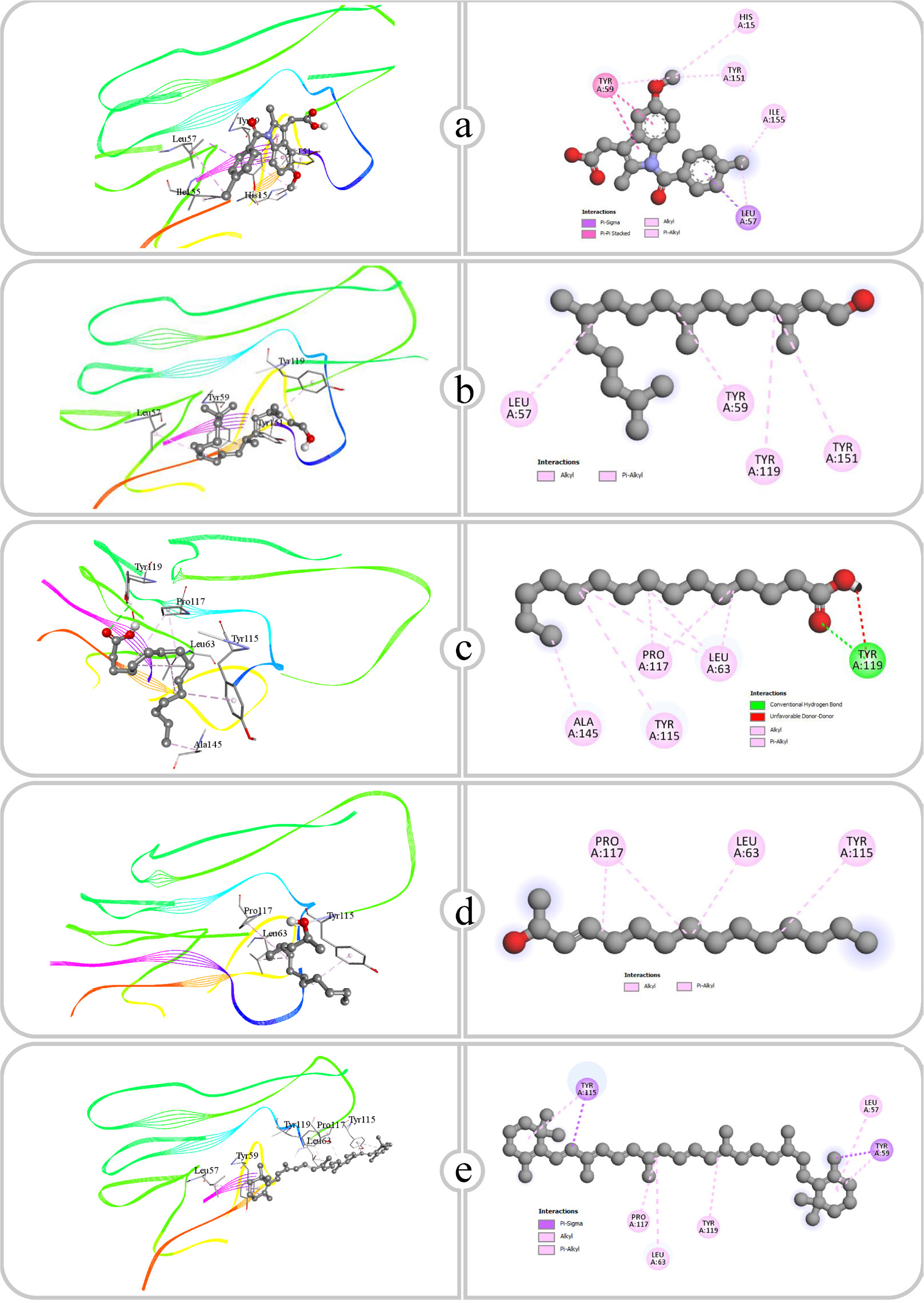
Figure 9:
Binding affinity and molecular interaction of (a) Indomethacin, (b) Phytol, (c) Pentadecanoic acid, (d) E-3-Pentadecen-2-ol and (e) β Carotene against TNF-α (2az5).
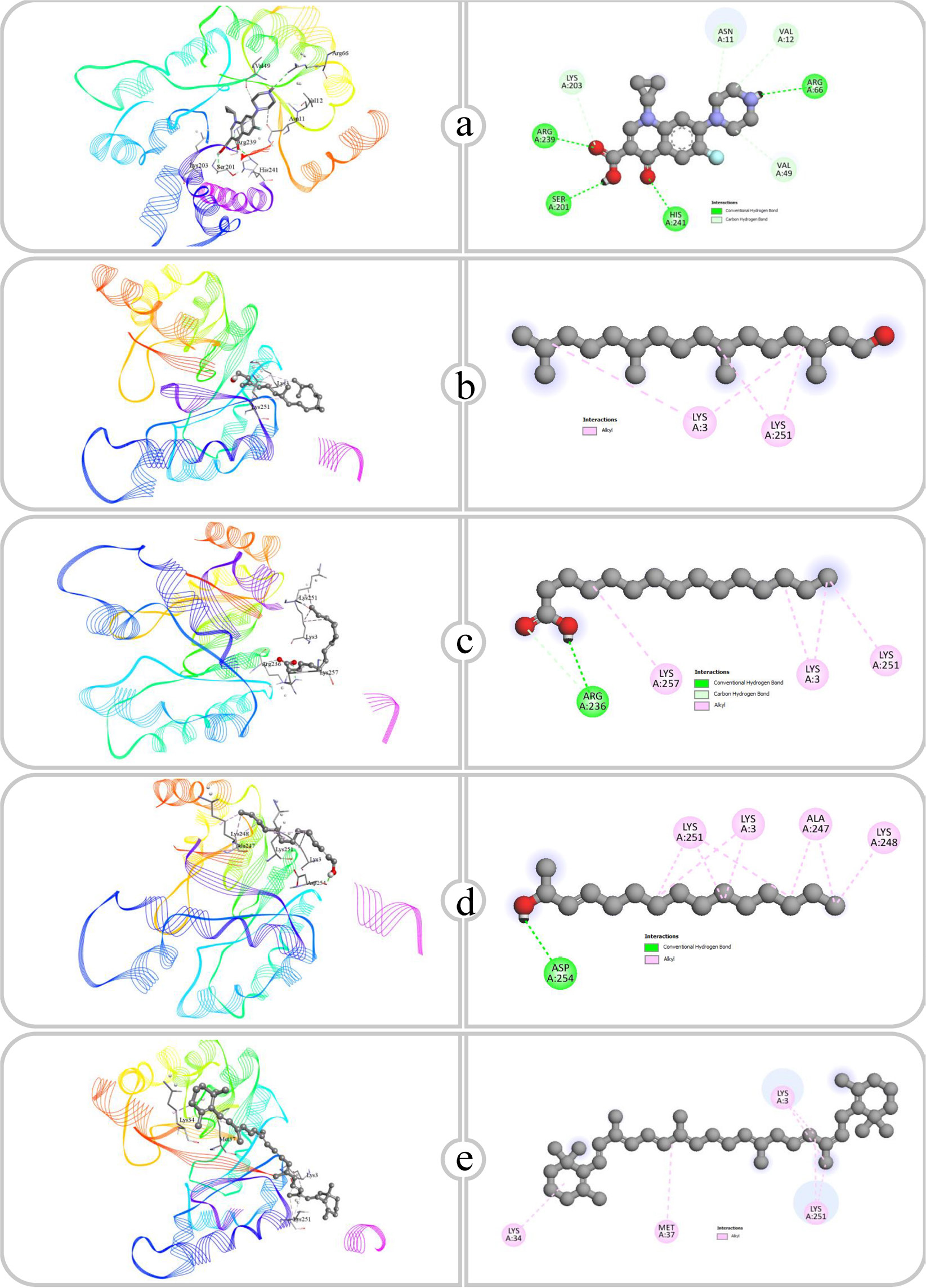
Figure 10:
Binding affinity and molecular interaction of (a) Ciprofloxacin, (b) Phytol, (c) Pentadecanoic acid, (d) E-3-Pentadecen-2-ol and (e) β Carotene against Dihydropteroate synthase (1ad4).
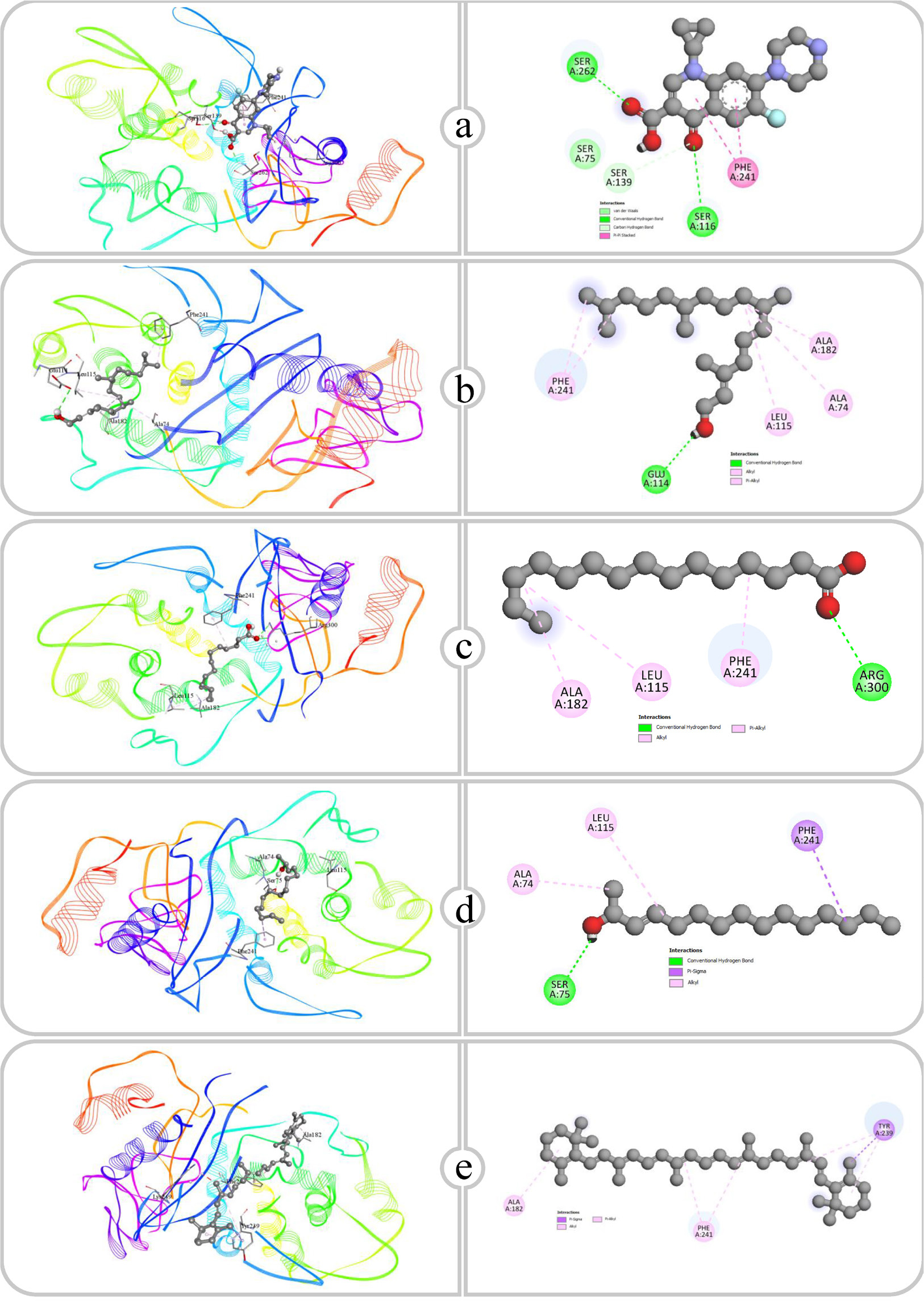
Figure 11:
Binding affinity and molecular interaction of (a) Ciprofloxacin, (b) Phytol, (c) Pentadecanoic acid, (d) E-3-Pentadecen-2-ol and (e) β Carotene against Transpeptidase (5tw8).
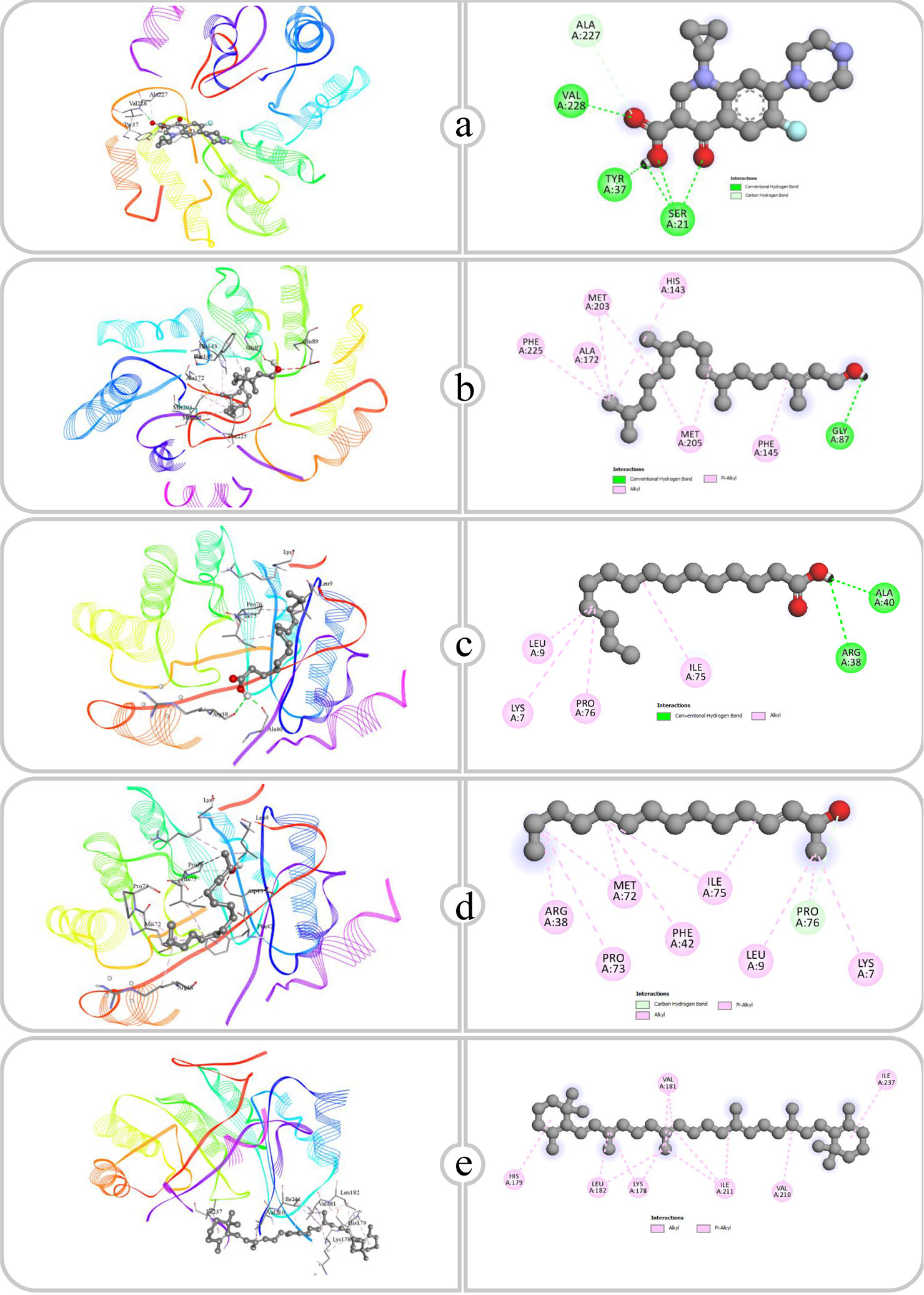
Figure 12:
Binding affinity and molecular interaction of (a) Ciprofloxacin, (b) Phytol, (c) Pentadecanoic acid, (d) E-3-Pentadecen-2-ol and (e) β Carotene against Type I dehydroquinase (4cno).
Sl. No. | Name of the compound | COX-2 (5f19) | IL-1β (4g6m) | NF-KAPPA (1nfi) | Phospholipase A2 (1dcy) | TNF-α (2az5) |
---|---|---|---|---|---|---|
1 | Indomethacin | -7.6 | -6.6 | -6.4 | -8.8 | -6.2 |
2 | Docosanoic acid, 1, 2, 3- propanetriyl ester | -4.7 | -3.6 | -4.3 | -3.2 | -3.4 |
3 | Octadecanal, 2-bromo- | -6.6 | -4.7 | -5.0 | -5.9 | -4.6 |
4 | Phytol | -4.8 | -4.5 | -4.7 | -5.8 | -4.4 |
5 | Pentadecanoic acid | -4.7 | -4.1 | -3.9 | -5.5 | -3.9 |
6 | Tristearin | -6.9 | -4.4 | -3.4 | -5.5 | -3.4 |
7 | E-3-Pentadecen-2-ol | -5.3 | -4.7 | -4.3 | -5.4 | -3.9 |
8 | β Carotene | -9.0 | -7.8 | -7.6 | -7.3 | -7.7 |
Docking score of the compounds against anti-inflammatory proteins (kcal/mol).
Sl. No. | Name of compound | Receptor and ID | Hydrogen bond | Hydrogen bond distance (Å) | Hydrophobic bond |
---|---|---|---|---|---|
1 | Indomethacin | COX-2 (5f19) | ARG120, GLU524 | 2.73, 2.15 | VAL89, TYR115, TYR115, ILE112, VAL116, TRP100, TYR355, VAL89, VAL116, VAL89 |
IL-1β (4g6m) | SER5, LEU62, LEU67, SER43 | 2.09, 2.55, 2.34, 3.59 | PRO91 | ||
NF-κB (1nfi) | GLN119 | 2.23 | GLN119, VAL91, SER42, ALA43, ALA43, VAL91 | ||
Phospholipase A2 (1dcy) | ASP48, GLY29 | 2.71, 2.54 | ALA17, CYS44, PHE5, LYS62, HIS6, TYR51, LEU2, LEU2, ALA18 | ||
TNF-α (2az5) | – | – | LEU57, TYR59, TYR59, LEU57, ILE155, HIS15, TYR59, TYR151 | ||
2 | Phytol | COX-2 (5f19) | CYS569, VAL572 | 2.51, 2.25 | MET261, ARG307, ARG311, LYS573, MET261 |
IL-1β (4g6m) | LEU134, THR79 | 2.51, 3.29 | PHE133, LYS77, PRO131, LYS74, MET130, PRO131, PHE133 | ||
NF-κB (1nfi) | ASN155 | 3.72 | ALA188, ALA192, ALA192, PHE184, PHE184 | ||
Phospholipase A2 (1dcy) | HIS27 | 2.16 | HIS47, LEU2, ALA17, CYS28, VAL30, CYS44, ALA94, CYS44, LEU2, PHE5, PHE5, PHE98 | ||
TNF-α (2az5) | – | – | LEU57, TYR59, TYR119, TYR151 | ||
3 | Pentadecanoic acid | COX-2 (5f19) | SER119, ARG120, ARG120 | 3.02, 2.47, 2.95 | PRO84, VAL89, VAL89, VAL89, LEU93, VAL116, ILE112, ILE92, LEU93, ILE92, TRP100 |
IL-1β (4g6m) | LEU26 | 2.94 | LYS77, PRO131, PRO131, PHE133, PHE133 | ||
NF-κB (1nfi) | ASN115, ASN115 | 2.49, 2.06 | ALA102, ALA102 | ||
Phospholipase A2 (1dcy) | HIS47, ASP48 | 1.95, 2.16 | ALA17, ALA17, CYS28, VAL30, CYS44, LEU2, LEU2, PHE5, PHE5, PHE98 | ||
TNF-α (2az5) | TYR119 | 3.01 | LEU63, PRO117, PRO117, ALA145, LEU63, LEU63, TYR115 | ||
4 | E-3-Pentadecen -2-ol | COX-2 (5f19) | – | – | ARG44, VAL46, LEU152, PRO153, LYS468, ARG469, LEU152, TYR130 |
IL-1β (4g6m) | – | – | PRO131, PHE133 | ||
NF-κB (1nfi) | ASP185 | 2.36 | LYS123, LEU154, LEU154, LYS123, LEU126 | ||
Phospholipase A2 (1dcy) | – | – | ALA17, VAL30, LEU2, LEU2, ILE9, PHE5, PHE5, HIS6, HIS47 | ||
TNF-α (2az5) | – | – | PRO117, PRO117, LEU63, TYR115 | ||
5 | β Carotene | COX-2 (5f19) | – | – | LYS79, HIS122, HIS122, PHE142 |
IL-1β (4g6m) | – | – | TYR24, PRO131, TYR24, PHE133 | ||
NF-κB (1nfi) | – | – | ALA188, ALA192, ALA192, LEU215, LYS218, LYS218, PHE184, PHE184 | ||
Phospholipase A2 (1dcy) | – | – | LEU2, ALA17, VAL30, LYS115, LYS115, PHE5, PHE23, PHE23 | ||
TNF-α (2az5) | – | – | TYR115, TYR59, LEU57, LEU63, PRO117, TYR59, TYR115, TYR119 |
Interaction of amino acid residues of the selected compounds.
Sl. No. | Name of the compound | Dihydropteroate synthase (1ad4) | Transpeptidase (5tw8) | Type I dehydroquinase (4cno) |
---|---|---|---|---|
1 | Ciprofloxacin | -7.0 | -7.4 | -7.2 |
2 | Docosanoic acid, 1, 2, 3-propanetriyl ester | -3.5 | -3.4 | -3.8 |
3 | Octadecanal, 2-bromo- | -5.7 | -5.1 | -5.8 |
4 | Phytol | -5.2 | -5.4 | -4.7 |
5 | Pentadecanoic acid | -4.7 | -5.1 | -4.6 |
6 | Tristearin | -3.5 | -4.3 | -4.7 |
7 | E-3-Pentadecen-2-ol | -4.9 | -4.6 | -4.5 |
8 | β Carotene | -7.7 | -7.8 | -7.7 |
Docking score of the compounds against antimicrobial proteins (kcal/mol).
Sl. No. | Name of compound | Receptor and ID | Hydrogen bond | Hydrogen bond distance (Å) | Hydrophobic bond |
---|---|---|---|---|---|
1 | Ciprofloxacin | Dihydropteroate synthase (1ad4) | ARG239, HIS241, ARG66, SER201, LYS203, VAL49, VAL12, ASN11 | 2.36, 2.31, 2.63, 2.32, 3.61, 3.51, 3.63, 3.54 | – |
Transpeptidase (5tw8) | SER116, SER262, SER139 | 2.93, 2.32 3.50 | PHE241, PHE241 | ||
Type I dehydroquinase (4cno) | SER21, SER21, VAL228, SER21, TYR37, ALA227 | 2.69, 1.76, 2.03, 2.24, 2.20, 3.27 | – | ||
2 | Phytol | Dihydropteroate synthase (1ad4) | – | – | LYS3, LYS3, LYS251, LYS251 |
Transpeptidase (5tw8) | GLU114 | 2.84 | ALA74, ALA182, LEU115, PHE241, PHE241 | ||
Type I dehydroquinase (4cno) | GLY87 | 2.29 | ALA172, MET203, MET205, MET205, MET203, HIS143, PHE145, PHE225 | ||
3 | Pentadecanoic acid | Dihydropteroate synthase (1ad4) | ARG236, ARG236 | 2.63, 3.47 | LYS3, LYS257, LYS3, LYS251 |
Transpeptidase (5tw8) | ARG300, ARG300 | 3.31, 2.79 | ALA182, LEU115, PHE241 | ||
Type I dehydroquinase (4cno) | ARG38, ALA40 | 2.89, 1.91 | LYS7, ILE75, PRO76, LEU9 | ||
4 | E-3-Pentadecen-2-ol | Dihydropteroate synthase (1ad4) | ASP254 | 1.98 | LYS3, LYS3, ALA247, ALA247, LYS251, LYS251, LYS251, LYS248 |
Transpeptidase (5tw8) | SER75 | 2.10 | PHE241, ALA74, LEU115 | ||
Type I dehydroquinase (4cno) | PRO76 | 3.57 | ARG38, PRO73, ILE75, LYS7, LEU9, PRO76, MET72, ILE75, MET72, PHE42 | ||
5 | β Carotene | Dihydropteroate synthase (1ad4) | – | – | LYS3, LYS34, MET37, LYS251, LYS3, LYS251 |
Transpeptidase (5tw8) | – | – | TYR239, ALA182, TYR239, TYR239, PHE241, PHE241 | ||
Type I dehydroquinase (4cno) | – | – | LYS178, LYS178, VAL181, LEU182, VAL210, ILE211, ILE211, ILE237, VAL181, LEU182, ILE211, HIS179 |
Interaction of amino acid residues of the selected compounds.
DISCUSSION
The Phytoconstituents identified with GC-MS analysis, several of which are documented to exhibit significant anti-inflammatory and antimicrobial activities. Phytol represented the height peak percentage at 59.91%. Phytol is well-documented for its remarkable anti-inflammatory activity, contributing significantly to the biological potential of EOUD (Islamet al., 2018). Furthermore, other identified compounds, including, 2-bromo-; Pentadecanoic acid; and β-Carotene, also exhibited notable anti-inflammatory activity. This comprehensive profile highlights the synergistic effects of these bioactive compounds in supporting the anti-inflammatory properties of EOUD.
The CIPE model is a well-known method for examining anti-inflammatory activity, as it triggers acute inflammation through the release of pro-inflammatory mediators such as histamine and prostaglandins. Anti-inflammatory agents, including plant-derived compounds, can reduce swelling by inhibiting these mediators (Winteret al., 1962). The anti-inflammatory potential of the essential oil of Urochloa distachya was evaluated using the CIPE model, which revealed significant results at a dose of 400 mg/kg body weight.
Salmonella typhi causes typhoid fever, a systemic infection that affects the gastrointestinal tract, leading to fever, abdominal pain and intestinal issues. Transmitted through contaminated food and water, it poses a significant risk in areas with poor sanitation. The rise of antibiotic-resistant strains of S. typhi highlights the need for alternative treatments, such as plant-based antimicrobials (Daburet al., 2007). Staphylococcus aureus is a major pathogen responsible for a variety of infections, including skin and soft tissue infections, pneumonia and sepsis. It can cause both mild and severe diseases, with the potential for antibiotic resistance, particularly Methicillin-Resistant S. aureus (MRSA), complicating treatment (Shittu and Lin, 2006). The antimicrobial activity of the essential oil of Urochloa distachya was evaluated against S. typhi and S. aureus, two pathogens responsible for a range of infections. The results demonstrated significant antimicrobial effects. These findings suggest that the essential oil contains bioactive compounds that disrupt bacterial cell membranes or inhibit bacterial growth. Further studies are needed to determine the Minimum Inhibitory Concentration (MIC) of this essential oil, providing a clearer understanding of its therapeutic efficacy.
In this study, in silico molecular docking was employed to investigate the binding affinity of the compounds, aiming to evaluate their potential anti-inflammatory activity. The analysis focused on pivotal protein targets involved in inflammatory pathways, including Cyclooxygenase-2 (COX-2), Interleukin-1β (IL-1β), Nuclear Factor kappa B (NF-κB), phospholipase A2 and Tumor Necrosis Factor-alpha (TNF-α). These proteins were selected based on their essential roles in regulating inflammation, pain and immune responses. The compounds selected for binding affinity evaluation included Phytol, Pentadecanoic acid, E-3-Pentadecen-2-ol and β-Carotene, identified through GC-MS analysis of EOUD. These bioactive compounds were selected for their potential anti-inflammatory properties. Furthermore, Indomethacin, a Nonsteroidal Anti-Inflammatory Drug (NSAID) that is widely recognized, was employed as a standard reference to evaluate and validate the binding efficacy of the tested compounds.
Cyclooxygenase-2 (COX-2), a key enzyme involved in the synthesis of prostaglandins, has been recognized as a valuable target for treating inflammation (Juet al., 2022). Hydrogen bonds are formed with functional groups like Hydroxyl (OH) and carboxyl, which interact with polar residues such as ARG, GLU, CYS and SER, whereas hydrophobic regions associated with nonpolar amino acids involving TYR, VAL, TRP, LEU and PHE, thereby stabilizing each compound within the COX-2 enzyme binding site. The carboxy group of indomethacin and the NH group of ARG120 form a hydrogen bond, while GLU (Glutamic Acid) A: 524 also forms a hydrogen bond through its carboxy group. Hydrophobic interactions involve TYR115, TRP100, VAL116 and VAL89, which interact with the benzene rings and other nonpolar regions of Indomethacin. Phytol primarily interacts with COX-2 via hydrophobic bonds, while also establishing hydrogen bonds. The Hydroxy (OH) group of phytol shows hydrogen bonds with CYS569 and VAL572, securing its attachment to the enzyme. Furthermore, the extended hydrophobic chain of phytol participates in nonpolar interactions with ARG 907. MET261, ARG A: 311 and LYS573. Pentadecanoic acid produces hydrogen bonds by its carboxy group, interacting with the NH groups of ARG A: 120 and SER119 within the active site. The hydrophobic, aliphatic chain of pentadecanoic acid associates with nonpolar residues, such as VAL A: 89, TRP A: 100, LEU A: 93, PRO84 and ILE112. E-3-Pentadecen-2-ol primarily relies on hydrophobic interactions within the COX-2 active site. The aliphatic chain and hydroxyl group engage with ARG44, ARG469, TYR130, LYS468, LEU152 and PRO153. Beta-carotene interacts with COX-2 only through hydrophobic interactions because of its polyene structure. With the compound’s extended conjugated double bonds, PHE142, PRO127, HIS122 and LYS95 participate in nonpolar interactions.
IL-1β is a pro-inflammatory cytokine associated with pain, inflammation and autoimmune disorders (Ren and Torres, 2008). The interactions show that each ligand’s binding interactions with IL-1β are significantly influenced by the type and presence of functional groups, including hydroxyl, carboxyl, alkyl and aromatic groups. Indomethacin formed hydrogen bonds through its carboxyl group with amino acids such as SER5, LEU62, LEU67 and SER43. The interactions of these hydrogen bonds, occurring at distances between 2.09 and 3.59 Å, are likely to contribute considerable binding stability. Furthermore, the aromatic ring in Indomethacin promoted hydrophobic Pi-Alkyl interactions with PRO91. Phytol exhibited hydrogen bond interactions through its hydroxyl group with LEU134 and THR79 at distances of 2.51 and 3.29 Å. The long hydrophobic alkyl chain of Phytol facilitated numerous hydrophobic interactions with residues including PHE133, LYS77, PRO131, LYS74 and MET130. Pentadecanoic acid, a saturated fatty acid featuring a carboxyl group, engaged this polar functional group to form a hydrogen bond with LEU26 at a distance of 2.94 Å. The lengthy alkyl chain established significant hydrophobic interactions with residues such as LYS77, PRO131 and PHE133, enhancing its binding via hydrophobic stabilization. E-3-Pentadecen-2-ol primarily engaged in hydrophobic interactions through its alkyl chain with PRO131 and PHE133. The alkene functional group likely improved its hydrophobic binding, indicating that E-3-Pentadecen-2-ol interacts mainly through nonpolar contacts within the IL-1β binding site. β-Carotene, a highly hydrophobic molecule featuring conjugated double bonds, interacted solely through hydrophobic interactions with TYR24, PRO131 and PHE133. The conjugated double bonds in the structure of β-Carotene improve its Pi-Alkyl interactions, allowing it to fit effectively within the hydrophobic pockets of IL-1β.
NF-κB plays a dual role in inflammation, exhibiting anti-inflammatory effects by directly suppressing the expression of pro-inflammatory genes and modulating the expression or activity of anti-inflammatory cytokines, such as Interleukin-10 (IL-10) (Lawrence, 2009). NF-κB regulates key target genes that are crucial in the development and progression of inflammation. As an inducible transcription factor, NF-κB, upon activation, initiates the transcription of various genes, thereby playing a pivotal role in the regulation of inflammatory responses (Liuet al., 2017). Indomethacin established a hydrogen bond with GLN119 at a distance of 2.23 Å. Furthermore, Indomethacin exhibited hydrophobic interactions with residues GLN119, VAL91, SER42 and ALA43, which likely enhanced its stable binding within the NF-κB active site. Phytol formed a hydrogen bond with ASN155 at a distance of 3.72 Å. The hydrophobic contacts of phytol involved multiple alanine residues, specifically ALA188 and ALA192, along with PHE184, indicating that its binding is predominantly sustained by nonpolar interactions. Pentadecanoic acid, a saturated fatty acid, exhibited two hydrogen bonds with ASN115 at distances of 2.49 and 2.06 Å. The robust hydrogen bonding, coupled with hydrophobic interactions with ALA102, indicates that Pentadecanoic acid can form strong linkages by both polar and nonpolar interactions. The existence of several hydrogen bonds with ASN115 suggests that this ligand may secure a strong fit within NF-κB, thereby influencing its activity. E-3-Pentadecen-2-ol created a hydrogen bond with ASP185 at a distance of 2.36 Å and exhibited hydrophobic interactions with LYS123, LEU154 and LEU126. The interplay of hydrogen bonding and hydrophobic contacts suggests that E-3-Pentadecen-2-ol can form a stable connection with NF-κB. The unsaturated alkyl chain may improve its affinity for the hydrophobic domains of NF-κB. β-Carotene, a predominantly nonpolar molecule, depended solely on hydrophobic interactions with several residues, including ALA188, ALA192, LEU215, LYS218 and PHE184. The conjugated structure of β-Carotene likely enhances Pi-Alkyl and alkyl interactions inside the hydrophobic areas of NF-κB.
Phospholipase A2 (PLA2) inhibitors are compounds that reduce the amount of arachidonic acid released from cell membranes by blocking the action of the PLA2 enzyme. These compounds reduce inflammation by inhibiting PLA2, which in turn decreases the synthesis of inflammatory mediators (Khan and Ilies, 2024). Indomethacin exhibited a significant binding affinity for Phospholipase A2 (PLA2) through essential interactions. The carboxylic acid group established hydrogen bonds with ASP48 (2.71 Å) and GLY29 (2.54 Å), which are essential for stabilizing the complex. Hydrophobic interactions involving residues such as PHE5, LEU2 and ALA17 strengthened the binding. The ASP48 interaction is significant for its role in positioning Indomethacin in the active site. Phytol shows notable interactions with the active site of the target protein, mainly through its functional groups and hydrophobic moieties. A significant hydrogen bond is established between the Hydroxyl group (-OH) of Phytol and HIS27, exhibiting a bond distance of 2.16 Å. The extended hydrophobic tail of Phytol interacts extensively with residues including ALA17, LEU2, VAL30 and PHE5 through various hydrophobic interactions. Pentadecanoic acid interacts effectively with the binding site of the target protein, showcasing critical hydrogen bonds and hydrophobic interactions. The Carboxyl group (-COOH) of Pentadecanoic acid forms two strong hydrogen bonds: one with HIS47 at a bond distance of 1.95 Å and another with ASP48 at 2.16 Å.
Tumor Necrosis Factor alpha (TNF-α) is a cytokine with pleiotropic effects on various cell types. It is recognized as a key regulator of inflammatory responses and plays a critical role in the pathogenesis of several inflammatory and autoimmune diseases (Janget al., 2021). The binding interaction of pentadecanoic acid with the TNF-α protein encompasses both hydrophobic and hydrogen bonding interactions. The alkyl chain of pentadecanoic acid participates in hydrophobic interactions with various residues, including ALA145, PRO117, LEU63 and TYR115. Additionally, a key hydrogen bond is formed between the carboxylic acid group of pentadecanoic acid and the hydroxyl group of Tyrosine (TYR119). This hydrogen bond interaction occurs at a distance of 3.01 Å, indicating a strong and specific interaction that enhances the binding affinity of pentadecanoic acid to TNF-α.
Molecular docking studies of antimicrobial compounds targeting Dihydropteroate Synthase (DHPS), transpeptidase and type I dehydroquinase indicate substantial potential for disrupting critical bacterial processes. Dihydropteroate Synthase (DHPS) is an essential bacterial enzyme involved in the synthesis of 7,8-dihydropteroate, a precursor in the folate biosynthesis pathway. This pathway results in the formation of tetrahydrofolate, crucial for nucleotide synthesis and bacterial proliferation. Antibacterial drugs inhibit Dihydropteroate Synthase (DHPS) by competing with its natural substrate, Para-Aminobenzoic Acid (PABA), obstructing folate synthesis and ultimately inhibiting bacterial proliferation (Kakkasseryet al., 2021). This study focuses on the binding of ciprofloxacin, phytol, pentadecanoic acid, E-3-pentadecen-2-ol and beta-carotene to DHPS with emphasis being made on the role of the functional groups in stabilizing these compounds within the DHPS active site. Considering these factors, ciprofloxacin is a fluoroquinolone class and can show multiple hydrogen bonding characteristics with the Carboxy (-COOH) and Amine (-NH) functional groups. These groups interacted with the amino acids, specifically ARG239, HIS241, AG66, SER202 and LYS203, at a distance of 2.31 and 3.63 Å. These hydrogen bonds are crucial for remarkable binding affinity. The phytol is a long-chain alcohol and absence of polar functional groups, mostly engages in hydrophobic interactions, with the aliphatic tail interacting with LYS3 and LYS51. This interaction is less stable than hydrogen bonding; however, it contributes to the stabilization of the compound within the non-polar regions of the enzyme. Pentadecanoic acid possessing one carboxyl group, facilitated hydrogen bonding with ARG236 at distances of 2.63 and 3.47 Å and was stabilized through hydrophobic interactions involving LYS3, LYS257 and LYS251. The interaction of hydrogen bonding through the carboxy group and hydrophobic stabilization from the aliphatic chain enables pentadecanoic acid to effectively bind to DHPS. E-3-Pentadecen-2-ol, which possesses a Hydroxyl (-OH) group and a double bond in its aliphatic chain, exhibited hydrogen bonding and hydrophobic interactions. The hydroxyl group formed a close hydrogen bind with ASP254 (1.98 Å), while the aliphatic chain interacted hydrophobically with LYS3, LYS251, ALA247 and LYS248, enabling dual binding that stabilizes the compound within DHPS. In contrast, the nonpolar molecule beta-carotene, characterized by its extended conjugated bonding, does not contain functional groups that can participate in hydrogen bonding. Therefore, its binding is completely dependent on hydrophobic interactions with LYS3, LYS34, MET37 and LYS251. The hydrophobic interaction is typically weaker than polar interactions; however, the extended conjugated chain of beta-carotene facilitates binding within the hydrophobic pockets of DHPS. The binding affinity of compounds to DHPS is predominantly determined by the characteristics of its functional groups. Polar functional groups such as -COOH and -OH promote hydrogen bonding and enhance interactions, as seen with ciprofloxacin and pentadecanoic acid.
Transpeptidase is a bacterial enzyme that strengthens the cell wall through the cross-linking of peptidoglycan. Antibacterial drugs inhibit this enzyme, obstructing cross-link production, hence weakening the cell wall and undermining bacterial integrity (Allison and Lambert, 2024). The affinity of each molecule for transpeptidase is dependent upon the functional groups present. Polar groups, represented by ciprofloxacin, phytol and pentadecanoic acid, promote hydrogen bonding, whereas nonpolar molecules such as E-3-Pentadecen-2-ol and β-carotene predominantly depend on hydrophobic interactions. Ciprofloxacin makes substantial hydrogen bonds with the amino acids SER116, SER262 and SER139, at distances of 2.93 Å, 2.32 Å and 3.50 Å, respectively. The hydrogen bonds probably link ciprofloxacin’s polar functional groups, including its carboxyl and ketone groups, which work as both hydrogen bond donors and acceptors. Ciprofloxacin also participates in hydrophobic interactions with PHE241. Phytol forms a hydrogen bond with GLU114 at a distance of 2.84 Å, likely involving its hydroxyl group. The hydrogen bond secures phytol in the binding site. Moreover, phytol showed several hydrophobic interactions with ALA74, ALA182, LEU115 and PHE241. Pentadecanoic Acid interacts with ARG300 through two hydrogen bonds, exhibiting bond lengths of 3.31 Å and 2.79 Å, respectively. The carboxyl group of pentadecanoic acid engages in strong hydrogen bonding with the positively charged guanidinium group of arginine, creating a stable polar interaction. In addition, pentadecanoic acid forms hydrophobic contacts with ALA182, LEU115 and PHE241, where its hydrocarbon chain fits into the nonpolar regions of the binding site, further stabilizing the binding through hydrophobic interactions. E-3-Pentadecen-2-ol possesses hydroxyl and aliphatic groups, enabling it to participate in hydrogen bonding and hydrophobic interactions. It forms a hydrogen bond to SER75 at an angle of 2.10 Å, likely through its hydroxyl group. E-3-Pentadecen-2-ol exhibits hydrophobic interactions with PHE241, ALA74 and LEU115, showing that its nonpolar carbon chain aligns with the hydrophobic side chains of these amino acids. The large conjugated hydrocarbon structure of β-carotene interacts with the hydrophobic amino acids TYR239, ALA182 and PHE241. The substantial, nonpolar structure of β-Carotene facilitates optimal van der Waals interactions within the binding site, ensuring an effective hydrophobic binding fit.
Type I dehydroquinases, adopting a Schiff base mechanism, serve as vital for the microbial and plant biosynthesis of folate, ubiquinone and aromatic amino acids. Their absence in animals renders them a suitable target for antibacterial drugs (Maneiroet al., 2014). The binding interactions of compounds with Type I dehydroquinase demonstrate various hydrogen bonding and hydrophobic interactions that enhance their stability in the active site. Ciprofloxacin forms hydrogen bonds with the residues SER21, VAL228, TYR37 and ALA227, with bond lengths between 1.76 Å and 3.27 Å. Phytol creates a hydrogen bond with GLY87 and exhibits significant hydrophobic interactions with residues such as ALA172, MET203 and HIS143. Pentadecanoic acid similarly forms hydrogen bonds with ARG38 and ALA40 and exhibits hydrophobic interactions with LYS7, ILE75 and LEU9. E-3-Pentadecen-2-ol makes a hydrogen bond with PRO76, while its nonpolar chain conforms to hydrophobic bonds, interacting with residues including ARG38, PRO73 and MET72. β-Carotene establishes significant hydrophobic contacts with the residues LYS178, VAL181, LEU182 and ILE211. These interactions show that the binding of each compound to Type I dehydroquinase is affected by its functional groups, with polar compounds establishing hydrogen bonds and nonpolar compounds relying mainly on hydrophobic interactions, emphasizing the varied mechanisms of association within the enzyme’s active site.
CONCLUSION
In conclusion, Urochloa distachya emerges as a promising plant with essential oils that demonstrate significant anti-inflammatory and antimicrobial activities. The GC-MS analysis revealed Phytol, an acyclic diterpenoid alcohol, as the major bioactive compound responsible for these effects. Phytol has been previously recognized for its anti-inflammatory and antimicrobial properties, which aligns with the observed outcomes in this study. The in vivo evaluation using the carrageenan-induced paw edema model further validated the anti-inflammatory potential of the essential oil, showing a significant reduction in inflammation at a dose of 400 mg/kg body weight. Additionally, the essential oil exhibited notable antibacterial activity against Salmonella typhi and Staphylococcus aureus, two pathogens responsible for severe infections, further supporting its therapeutic potential. Moreover, in silico molecular docking studies provided further insights into the binding affinity of the phytoconstituents identified through GC-MS, offering a deeper understanding of their mechanisms of action. The results from this study highlight the plant’s promise as a natural source of bioactive compounds with pharmacological benefits. Nevertheless, additional research is required to comprehensively investigate the phytochemical profile and pharmacological mechanisms of Urochloa distachya to fully explore its clinical applications and therapeutic potential.
Cite this article:
Dash S, Sidhan R. Anti-Inflammatory and Antimicrobial Activities of Essential Oil of Urochloa distachya (L.). Int. J. Pharm. Investigation. 2025;15(3):313-24.
REFERENCES
- Ábrego-García, A., Poggi-Varaldo, H. M., Ponce-Noyola, M. T., Calva-Calva, G., Galíndez-Mayer, C. J. J., Medina-Mendoza, G. G., & Rinderknecht-Seijas, N. F. (2022). Bioprocessing of two crop residues for animal feeding into a high-yield lovastatin feed supplement. Animals: An Open Access Journal from MDPI, 12(19), Article 19. https://doi.org/10.3390/ani12192697
- Alexander, J. A. N., Chatterjee, S. S., Hamilton, S. M., Eltis, L. D., Chambers, H. F., & Strynadka, N. C. J. (2018). Structural and kinetic analyses of penicillin-binding protein 4 (PBP4)-mediated antibiotic resistance in Staphylococcus aureus. Journal of Biological Chemistry, 293(51), 19854–19865. https://doi.org/10.1074/jbc.RA118.004952
- Allison, D. G., & Lambert, P. A. (2024). Modes of action of antibacterial agents. In Y.-W. Tang, M. Y. Hindiyeh, D. Liu, A. Sails, P. Spearman, & J.-R. Zhang (Eds.), Molecular medical microbiology (3rd ed.) (pp. 597–614). Academic Press. https://doi.org/10.1016/B978-0-12-818619-0.00133-7
- Anonymous. (2001). OECD Guideline for testing of Chemicals. Acute oral toxicity-acute toxic class method. Guideline 423.
- Antimicrobial Resistance Collaborators. (2022). Global burden of bacterial antimicrobial resistance in 2019: A systematic analysis. The Lancet, 399(10325), 629–655. https://doi.org/10.1016/S0140-6736(21)02724-0
- Anyasor, G. N., Okanlawon, A. A., & Ogunbiyi, B. (2019). Evaluation of anti-inflammatory activity of Justicia secunda Vahl leaf extract using in vitro and in vivo inflammation models. Clinical Phytoscience, 5(1), 49. https://doi.org/10.1186/s40816-019-0137-8
- Azab, A., Nassar, A., & Azab, A. N. (2016). Anti-inflammatory activity of natural products. Molecules, 21(10), 1321. https://doi.org/10.3390/molecules21101321
- Berg, G., Rybakova, D., Fischer, D., Cernava, T., Vergès, M.-C. C., Charles, T., Chen, X., Cocolin, L., Eversole, K., Corral, G. H., Kazou, M., Kinkel, L., Lange, L., Lima, N., Loy, A., Macklin, J. A., Maguin, E., Mauchline, T., McClure, R., . . . Schloter, M. (2020). Microbiome definition re-visited: Old concepts and new challenges. Microbiome, 8(1), 103. https://doi.org/10.1186/s40168-020-00875-0
- Blech, M., Peter, D., Fischer, P., Bauer, M. M. T., Hafner, M., Zeeb, M., & Nar, H. (2013). One target-two different binding modes: Structural insights into gevokizumab and canakinumab interactions to interleukin-1β. Journal of Molecular Biology, 425(1), 94–111. https://doi.org/10.1016/j.jmb.2012.09.021
- Chan, P., Cheng, J. T., Tsao, C. W., Niu, C. S., & Hong, C. Y. (1996). The in vitro antioxidant activity of trilinolein and other lipid-related natural substances as measured by enhanced chemiluminescence. Life Sciences, 59(24), 2067–2073. https://doi.org/10.1016/s0024-3205(96)00560-7
- Charles-Messance, H., Mitchelson, K. A. J., De Marco Castro, E., Sheedy, F. J., & Roche, H. M. (2020). Regulating metabolic inflammation by nutritional modulation. The Journal of Allergy and Clinical Immunology, 146(4), 706–720. https://doi.org/10.1016/j.jaci.2020.08.013
- Dabur, R., Gupta, A., Mandal, T. K., Singh, D. D., Bajpai, V., Gurav, A. M., & Lavekar, G. S. (2007). Antimicrobial activity of some Indian medicinal plants. African Journal of Traditional, Complementary, and Alternative Medicines, 4(3), Article 3. https://doi.org/10.4314/ajtcam.v4i3.31225
- Dash, S., Bohidar, J., Das, C., Mohanty, A., Meher, A., & Hota, R. (2023). Evaluation of anthelmintic activity and GC-MS characterization of Urochloa distachya (L.). International Journal of Pharmaceutical Investigation, 13(2), Article 2. https://doi.org/10.5530/ijpi.13.2.034
- Dash, S., Meher, A., Das Dash, S. K., C., & Dash, S. K. (2022). GC-MS analysis of methanolic extract of Urochloa distachya (L.) T. Q. Nguyen, leave| (6). 13, 6, Article 6. https://doi.org/DOI. https://doi.org/10.13040/IJPSR.0975-8232.13(1).2380-94
- Dash, S., Nanda, N., Bhanja, M., Sao, R. B., Roy, A., & Dash, R. (2024). GC-MS and molecular docking analyses of phytoconstituents from the plant Tephrosia purpurea. Journal of Chemical Health Risks, 14(5), Article 5. https://doi.org/10.52783/jchr.v14.i5.6071
- Dinesh Kumar, G., Karthik, M., & Rajakumar, R. (2018). GC-MS analysis of bioactive compounds from ethanolic leaves extract of Eichhornia crassipes (Mart.) Solms. And their pharmacological activities, 2018, 7(8), Article 8.
- Geetha, D. H., Rajeswari, M., & Jayashree, I. (2013). Chemical profiling of Elaeocarpus serratus L. by GC-MS. Asian Pacific Journal of Tropical Biomedicine, 3(12), Article 12. https://doi.org/10.1016/S2221-1691(13)60190-2
- Hampele, I. C., D’Arcy, A., Dale, G. E., Kostrewa, D., Nielsen, J., Oefner, C., Page, M. G. P., Schönfeld, H. J., Stüber, D., & Then, R. L. (1997). Structure and function of the dihydropteroate synthase from staphylococcus aureus 1. Journal of Molecular Biology, 268(1), 21–30. https://doi.org/10.1006/jmbi.1997.0944
- He, M. M., Smith, A. S., Oslob, J. D., Flanagan, W. M., Braisted, A. C., Whitty, A., Cancilla, M. T., Wang, J., Lugovskoy, A. A., Yoburn, J. C., Fung, A. D., Farrington, G., Eldredge, J. K., Day, E. S., Cruz, L. A., Cachero, T. G., Miller, S. K., Friedman, J. E., Choong, I. C., & Cunningham, B. C. (2005). Small-molecule inhibition of TNF-α. Science, 310(5750), 1022–1025. https://doi.org/10.1126/science.1116304
- Ify, O. A., Raphael, A. G., Tochukwu, O. C., Amarachi, O. U. S., Ikechukwu, N. A., Madukaihe, M. J., Thomas, Y., & Innocent, O. C. (2021). The antimicrobial, anti inflammatory and analgesic activities of the rhizome extract of Curcuma longa L. (turmeric). Journal of Advances in Biology and Biotechnology, 1–16. https://doi.org/10.9734/jabb/2021/v24i630217
- Islam, M., Ali, E., Uddin, S., Shaw, S., & Islam, M. A., Ahmed, Md. I., Shill, M. Karmakar, U., Yarla, N. sastry, Khan, I., Billah. MM, G., Malainer, C., Nicoletti, F., Gulei, D., Berindan-Neagoe, I., Apostolov, A., Banach, M. and Atanasov, A. (2018). Phytol: A review of biomedical activities. Food and Chemical Toxicology, 121. https://doi.org/10.1016/j.fct.2018.08.032.
- Jacobs, M. D., & Harrison, S. C. (1998). Structure of an IκBα/NF-κB complex. Cell, 95(6), 749–758. https://doi.org/10.1016/S0092-8674(00)81698-0
- Jang, D.-I., Lee, A.-H., Shin, H.-Y., Song, H.-R., Park, J.-H., Kang, T.-B., Lee, S.-R., & Yang, S.-H. (2021). The role of tumor necrosis factor alpha (TNF-α) in autoimmune disease and current TNF-α inhibitors in therapeutics. International Journal of Molecular Sciences, 22(5), Article 5. https://doi.org/10.3390/ijms22052719
- Ju, Z., Li, M., Xu, J., Howell, D. C., Li, Z., & Chen, F.-E. (2022). Recent development on COX-2 inhibitors as promising anti-inflammatory agents: The past 10 years. Acta Pharmaceutica Sinica. B, 12(6), 2790–2807. https://doi.org/10.1016/j.apsb.2022.01.002
- K, R., Kakkassery, J. T., Raphael, V. P., Johnson, R., & K, V. T. (2021). In vitro antibacterial and in silico docking studies of two Schiff bases on Staphylococcus aureus and its target proteins. Future Journal of Pharmaceutical Sciences, 7(1), 78. https://doi.org/10.1186/s43094-021-00225-3
- Kaur, S., Sharma, P., Bains, A., Chawla, P., Sridhar, K., Sharma, M., & Inbaraj, B. S. (2022). Antimicrobial and anti-inflammatory activity of low-energy assisted nanohydrogel of Azadirachta indica oil. Gels, 8(7), Article 7. https://doi.org/10.3390/gels8070434
- Khan, S. A., & Ilies, M. A. (2024). Phospholipases A2. In C. T. Supuran & W. A. Donald (Eds.), Metalloenzymes, A2 (pp. 101–136). Academic Press. https://doi.org/10.1016/B978-0-12-823974-2.00018-8
- Khandelwal, K. (2007). Practical pharmacognosy. Nirali Prakashan.
- Kováč, J., Slobodníková, L., Trajčíková, E., Rendeková, K., Mučaji, P., Sychrová, A., & Bittner Fialová, S. (2022). Therapeutic potential of flavonoids and tannins in management of oral infectious diseases-A review. Molecules, 28(1), Article 1. https://doi.org/10.3390/molecules28010158
- Lawrence, T. (2009). The nuclear factor NF-κB pathway in inflammation. Cold Spring Harbor Perspectives in Biology, 1(6), Article a001651. https://doi.org/10.1101/cshperspect.a001651
- Liu, T., Zhang, L., Joo, D., & Sun, S.-C. (2017). NF-κB signaling in inflammation. Signal Transduction and Targeted Therapy, 2(1), Article 17023. https://doi.org/10.1038/sigtrans.2017.23
- Loizou, S., Lekakis, I., Chrousos, G. P., & Moutsatsou, P. (2010). Beta-sitosterol exhibits anti-inflammatory activity in human aortic endothelial cells. Molecular Nutrition and Food Research, 54(4), 551–558. https://doi.org/10.1002/mnfr.200900012
- Lucido, M. J., Orlando, B. J., Vecchio, A. J., & Malkowski, M. G. (2016). Crystal structure of aspirin-acetylated human cyclooxygenase-2: Insight into the formation of products with reversed stereochemistry. Biochemistry, 55(8), 1226–1238. https://doi.org/10.1021/acs.biochem.5b01378
- Maneiro, M., Peón, A., Lence, E., Otero, J. M., Van Raaij, M. J., Thompson, P., Hawkins, A. R., & González-Bello, C. (2014). Insights into substrate binding and catalysis in bacterial type I dehydroquinase. The Biochemical Journal, 462(3), 415–424. https://doi.org/10.1042/BJ20140614
- Marchesi, J. R., & Ravel, J. (2015). The vocabulary of microbiome research: A proposal. Microbiome, 3(1), 31. https://doi.org/10.1186/s40168-015-0094-5
- Medzhitov, R. (2008). Origin and physiological roles of inflammation. Nature, 454(7203), 428–435. https://doi.org/10.1038/nature07201
- Medzhitov, R. (2010). Inflammation 2010: New adventures of an old flame. Cell, 140(6), 771–776. https://doi.org/10.1016/j.cell.2010.03.006
- Nisa, S., Shoukat, M., Bibi, Y., Al Ayoubi, S., Shah, W., Masood, S., Sabir, M., Asma Bano, S., & Qayyum, A. (2022). Therapeutic prospects of endophytic Bacillus species from Berberis lycium against oxidative stress and microbial pathogens. Saudi Journal of Biological Sciences, 29(1), Article 1. https://doi.org/10.1016/j.sjbs.2021.08.099
- Okoye, N. N., Ajaghaku, D. L., Okeke, H. N., Ilodigwe, E. E., Nworu, C. S., & Okoye, F. B. C. (2014). Beta-amyrin and alpha-amyrin acetate isolated from the stem bark of Alstonia boonei display profound anti-inflammatory activity. Pharmaceutical Biology, 52(11), 1478–1486. https://doi.org/10.3109/13880209.2014.898078
- Panda, S. S., Sahoo, K., Rana, M., Rout, N. C., & Dhal, N. K. (2014). Antimicrobial activities and phytochemical investigation of some native Pteridophytes, 7(1), 43–45.
- Ren, K., & Torres, R. (2009). Role of interleukin-1β during pain and inflammation. Brain Research Reviews, 60(1), 57–64. https://doi.org/10.1016/j.brainresrev.2008.12.020
- Salvatori, E. S., Morgan, L. V., Ferrarini, S., Zilli, G. A. L., Rosina, A., Almeida, M. O. P., Hackbart, H. C. S., Rezende, R. S., Albeny-Simões, D., Oliveira, J. V., Gasparetto, A., Müller, L. G., & Dal Magro, J. (2023). Anti-inflammatory and antimicrobial effects of Eucalyptus spp. essential oils: A potential valuable use for an industry byproduct. Evidence-Based Complementary and Alternative Medicine: eCAM, 2023, Article 2582698. https://doi.org/10.1155/2023/2582698
- Schevitz, R. W., Bach, N. J., Carlson, D. G., Chirgadze, N. Y., Clawson, D. K., Dillard, R. D., Draheim, S. E., Hartley, L. W., Jones, N. D., Mihelich, E. D., Olkowski, J. L., Snyder, D. W., Sommers, C., & Wery, J.-P. (1995). Structure-based design of the first potent and selective inhibitor of human non-pancreatic secretory phospholipase A2. Nature Structural Biology, 2(6), 458–465. https://doi.org/10.1038/nsb0695-458
- Shittu, A. O., & Lin, J. (2006). Antimicrobial susceptibility patterns and characterization of clinical isolates of Staphylococcus aureus in KwaZulu-Natal Province, South Africa. BMC Infectious Diseases, 6(1), 125. https://doi.org/10.1186/1471-2334-6-125
- Singh, B., & Sharma, R. A. (2015). Anti-inflammatory and antimicrobial properties of flavonoids from Heliotropium subulatum exudate. Inflammation & Allergy Drug Targets, 14(2), 125–132. https://doi.org/10.2174/1871528114666160105113155
- Singh, M., Kumar, V., Singh, I., Gauttam, V., & Kalia, A. N. (2010). Anti-inflammatory Activity of Aqueous extract of Mirabilis jalapa Linn. Leaves. Pharmacognosy Research, 2(6), 364–367. https://doi.org/10.4103/0974-8490.75456
- Souto, A. L., Tavares, J. F., da Silva, M. S., Diniz, M. de F. F. M., de Athayde-Filho, P. F., & Barbosa Filho, J. M. B. (2011). Anti-inflammatory activity of alkaloids: An update from 2000 to 2010. Molecules, 16(10), 8515–8534. https://doi.org/10.3390/molecules16108515
- Tacconelli, E., Carrara, E., Savoldi, A., Harbarth, S., Mendelson, M., Monnet, D. L., Pulcini, C., Kahlmeter, G., Kluytmans, J., Carmeli, Y., Ouellette, M., Outterson, K., Patel, J., Cavaleri, M., Cox, E. M., Houchens, C. R., Grayson, M. L., Hansen, P., Singh, N., . . . World Health Organization Pathogens Priority List Working Group. (2018). Discovery, research and development of new antibiotics: The WHO priority list of antibiotic-resistant bacteria and tuberculosis. The Lancet. Infectious Diseases, 18(3), 318–327. https://doi.org/10.1016/S1473-3099(17)30753-3
- Thitinarongwate, W., Nimlamool, W., Khonsung, P., Mektrirat, R., & Kunanusorn, P. (2022). Anti-inflammatory Activity of Essential Oil from Zingiber ottensii Valeton in Animal Models. Molecules, 27(13), Article 13. https://doi.org/10.3390/molecules27134260
- Venn-Watson, S., Lumpkin, R., & Dennis, E. A. (2020). Efficacy of dietary odd-chain saturated fatty acid pentadecanoic acid parallels broad associated health benefits in humans: Could it be essential? Scientific Reports, 10(1), 8161. https://doi.org/10.1038/s41598-020-64960-y
- Viet, T. D., Xuan, T. D., & Anh, H. (2021). α-amyrin and β-amyrin Isolated from Celastrus hindsii Leaves and Their Antioxidant, Anti-Xanthine oxidase and Anti-Tyrosinase Potentials. Molecules, 26(23), Article 23. https://doi.org/10.3390/molecules26237248
- Winter, C. A., Risley, E. A., & Nuss, G. W. (1962). Carrageenin-induced edema in hind paw of the rat as an assay for anti-inflammatory drugs. Proceedings of the Society for Experimental Biology and Medicine. Society for Experimental Biology and Medicine, 111, 544–547. https://doi.org/10.3181/00379727-111-27849
- World Health Organization. (2019). No time to Wait: Securing the future from drug-resistant infections. https://www.who.int/publications/i/item/no-time-to-wait-securing-the-future-from-drug-resistant-infections
- Wu, S., Chen, R., Chen, J., Yang, N., Li, K., Zhang, Z., & Zhang, R. (2023). Study of the anti-inflammatory mechanism of β-carotene based on network pharmacology. Molecules, 28(22), Article 22. https://doi.org/10.3390/molecules28227540
- Yadav, R. P., Huo, C., Budhathoki, R., Budthapa, P., Bhattarai, B. R., Rana, M., Kim, K. H., & Parajuli, N. (2024). Antibacterial, antifungal and cytotoxic effects of endophytic Streptomyces species isolated from the Himalayan regions of Nepal and their metabolite study. Biomedicines, 12(10), Article 10. https://doi.org/10.3390/biomedicines12102192
- Yan, Y., Li, X., Zhang, C., Lv, L., Gao, B., & Li, M. (2021). Research progress on antibacterial activities and mechanisms of natural alkaloids: A review. Antibiotics, 10(3), 318. https://doi.org/10.3390/antibiotics10030318
57.Yang, W., Chen, X., Li, Y., Guo, S., Wang, Z., & Yu, X. (2020). Advances in pharmacological activities of terpenoids. Natural Product Communications, 15(3). https://doi.org/10.1177/1934578X20903555