ABSTRACT
In recent years, biomedical research has resulted in innovative methods for precisely altering genomes, advancing personalized diagnostics and therapeutics through genetic engineering. The Clustered Regularly Interspaced Short Palindromic Repeat technique (CRISPR) is one of these techniques that have been developed to combat bacteria, fungi, parasites, viruses and genetic abnormalities. Biosensors based on CRISPR/Cas have traditionally been used to detect nucleic acids, but their potential to detect small molecules and disease-associated proteins has yet to be explored. Recent discoveries about genes that bind to nucleic acids have revolutionized genome editing. Despite this, challenges remain with current genetic engineering techniques, including viral vector reliance, immunogenicity, insertion oncogenesis and retention of transgenes. Using tailored polymeric nanoparticles as delivery vehicles for CRISPR/Cas9 components provides promising avenues for improving safety and efficacy. Using a combined nanomedicine approach, nanotechnology integration and CRISPR/Cas systems may open new therapeutic avenues, enabling early disease diagnosis and selective therapy.
INTRODUCTION
Infectious diseases, such as HIV/AIDS, are incurable due to the frequency and specificity of outbreaks. Chronic illnesses such as cancer also claim thousands of lives every year.1 In today’s healthcare environment, cancer treatment is one of the biggest challenges.2 There are nearly 6 deaths for every 6 people, as a result of its high mortality and rapid spread, making it a life-threatening disease for humans worldwide. In 2020, nearly 10 million people will die from it and 19.3 million new cases are expected worldwide.3 Aside from causing death worldwide, infectious diseases are India’s third leading cause of death. In terms of globally prevalent diseases, HIV/AIDS, malaria, viral hepatitis, tuberculosis, diarrheal disorders and acute lower respiratory tract infections are among the most prevalent.4 Chemotherapy, radiotherapy and surgery are common methods used to treat cancer, but they are limited in their efficacy and are associated with severe side effects. In spite of this, medical technology advances have led to more sophisticated approaches, such as Photodynamic Therapy (PDT), gene therapy, immunotherapy and Photothermal Therapy (PTT). Their advanced capabilities and favorable characteristics have made these innovative modalities increasingly popular.5
Diagnostics and treatment modalities must be improved due to the persistent presence of infectious diseases and inadequate medical infrastructure. Viable populations continue to be harmed by lack of diagnostic tools and treatment options for infectious ailments. Hence, conventional and alternative therapies must be substantially enhanced.6 Molecular biomarkers such as proteins assist in the early diagnosis of disease, the monitoring of treatment and the assessment of prognosis. In addition to nucleic acid sequence amplification, immunological approaches that combine antigen detection and visualization offer enhanced diagnostic precision.7 Diagnostic methods for infectious diseases include Polymerase Chain Reaction (PCR) and extraction of pathogenic nucleic acids from biological samples.8
Diagnostic procedures are typically based on identifying the disease’s target sequence, cleaving it and reading the resulting signal. This is the theory that nucleic acids are desirable biomarkers for illness.9 Molecular manipulation and editing techniques have advanced significantly in the field of genetic engineering in recent years, providing biologists with precise methods to modify nearly any organism’s genome DNA to incorporate desired mutations, such as extensions, corrections, replacements and excisions, allowing for faster discovery and development of therapeutics for unusual genetic disorders.10,11 Researchers can study gene expression and control in vitro (cells) and in vivo (animals). Future regenerative therapies may treat genetic diseases and infectious diseases.12
CRISPR, TALEN, ZFNs, HEs and Cas9 are programmable nucleases that can be used to target specific genes.13
Adaptable immunity against invasive genetic material can be acquired by prokaryotes through the CRISPR/Cas systems. Nucleic acid spacers from invading genetic elements act as a memory device inside the genomic locus of CRISPR. The Cas enzyme then uses these sequences to target and eliminate foreign invaders. System-level interventions and adaptive mechanisms distinguish CRISPR/Cas systems at the molecular level.14 It was first described in 2016 that the CRISPR/Cas technique could be applied to molecular diagnostics to identify nuclear acids. A variety of effective methods have been developed using CRISPR/ Cas to detect and diagnose infectious and non-infectious diseases.15 There are congenital traits associated with genetic disorders, just as there are with infectious illnesses. These conditions are caused by mutations in DNA due to biochemical, physiological, or genetic factors. Viral-mediated transgenic expression and RNA interference have traditionally been used to treat these genetic diseases.16
Drug resistance may result from various factors such as patient-specific side effects, contraindications and the infectious nature of the condition. In addition, pathogen specificity may hinder the effectiveness of novel drugs, potentially resulting in dosage and administration issues. Drug administration for long periods of time or excessively can lead to increased side effects and toxicity, leading to further treatment complications.17 CRISPR technology has faced a number of significant challenges, including the efficient delivery of CRISPR components to target cells. The CRISPR system’s non-specific targeting of nucleic acids has raised concerns, particularly given its intended use for diagnostic and therapeutic purposes.18 For CRISPR/Cas enzymes to work successfully inside genes, they must reach your desired cells and tissues without off-target effects. A number of researchers are investigating off-target difficulties, either by developing off-target detection methods or by developing CRISPR tools.
CRISPR/Cas 9
The genome of microorganisms is protected against damage caused by bacteriophage infections and plasmid transfer through a variety of mechanisms. A RNA-based defense system provided acquired immunity against viral and plasmid attacks by Eubacteria and Archaea recognizes and destroys external DNA and RNA. CRISPR-associated (Cas) 9 enzymes are RNA-driven endonucleases used as a defensive technique.19–21 Microorganisms can detect and eliminate foreign genetic material by using the CRISPR/Cas9 system in prokaryotes. Figure 1 highlights the integration of CRISPR-Cas systems with various nanocarriers, showcasing their potential to revolutionize personalized medicine by enhancing the specificity and efficiency of therapeutic interventions. There are many prokaryotic genomes with this enzyme and it was first detected in Escherichia coli in 1987. An irregular genomic array with “spacer’s” interspersed between similar sequences distinguishes this system from duplicated ones.22 By detecting and protecting themselves from genomic invaders, microbes activate defense mechanisms when they are exposed to foreign genetic material through processes such as transformation, conjugation, or transduction.23 Figure 2 outlines the hurdles in therapeutic applications, such as off-target effects and delivery system limitations, while also highlighting advancements that are addressing these issues, paving the way for safer and more effective clinical outcomes.
CRISPR-Cas functional ability
DNA invading organisms are identified and hydrolyzed by the CRISPR/Cas system.24 A three-stage mechanism can be described as follows: (1) adaptation, (2) crRNA maturation and (3) target interference.
Adaptation or spacer acquisition
This stage involves integrating the spacer into the CRISPR array once foreign genetic elements have been identified. In addition to retaining a piece of the genetic code of the intruder, a protospacer sequence is chosen and examined, demonstrating the adaptive nature of the immune system.25 As a result of this phase, the genetic memory is formed where re-invading nucleic acids can be neutralized by expressing and interrupting subsequent nucleic acids.26 For some CRISPR/Cas types, collecting protospacers and processing them before integrating them is still a work in progress. Biochemistry of the integration of spacers has been revealed by recent discoveries, on the other hand.27 Once the spacer has been identified in size, its integration into the host genome will be determined by the presence of the Integrated Host Factor (IHF). A nuclease protein is one of the most important components of the CRISPR/Cas complex. A dimer of these two proteins identifies foreign DNA by interacting together. Nuclear integrases, such as Cas1, are able to cleave bacterial DNA into spacers, while endoribonucleases, such as Cas2, cleave RNAs.28
Maturation
Invader sequences are deciphered from mature reference crRNAs derived from the CRISPR series.29 There is no consistent pattern for identifying CRISPR/Cas loci in these organisms, but RNA-protein complexes typically form after CRISPR/Cas loci are transcribed. In both forms of CRISPR, transcription takes place and the Casribonucleases process the RNA into the CRISPR ribonucleoprotein complex (crRNP).30 A single spacer and partial repeats are found in each component of the pre-crRNA after it has been cleaved. RNAse III, encoded outside of CRISPR loci, catalyzes this process of cleavage. Furthermore, tracrRNA, another complementary type of RNA, plays an important role in this process.31 CRRNA processing and crRNP complex formation are similar in type I and type III CRISPR/Cas systems. For Type I and III systems, instead of Cas5d, Cas6 protein processes pre-crRNA.32
Interference
CrRNA matures and triggers the interference cascade complex, which identifies nucleotide sequences that resemble packed crRNA. R-loop formation makes it possible to cleave each strand of invading genomic DNA.33 The 3′-5′ endonuclease of Cas3 assists in the cleavage of the target DNA and the subsequent degradation of the DNA. A spacer sequence containing 7- to 8-nucleotides at its 5′ end directs homology coupling with target sequences and causes R-loops to form. Prospacer Adjacent Motif (PAM) is identified before the target protospacer in order to complete this process.34,35
DNA biosensors for molecular diagnostics
For molecular diagnostic techniques, nucleic acids are an important target for detecting infectious diseases, antimicrobial resistance genes, cancer mutations, genetic disease mutations, Single Nucleotide Polymorphisms (SNPs) and biomarkers.36,37 The amplification step is usually the defining characteristic of conventional molecular diagnostic methods. Detecting gene mutations and identifying microbial species are the primary goals of Fluorescent in situ Hybridization (FISH), a non-amplification cytogenetic technique. Fluorescence microscopy enables our method to detect the presence of specific complementary target sequences by hybridizing synthetic fluorochrome-labeled oligonucleotides with them. Chromosome changes associated with cancers such as Leukemia, myelodysplastic syndromes and multiple myeloma are detected by FISH. Labeled oligonucleotide probe availability limits this technique, despite its time-consuming nature. Additionally, fluorescent microscopes have a high Limit of Detection (LOD), which necessitates an amplification step.38 Clinical molecular laboratories routinely use amplification-based molecular techniques as the “gold standard.” As a result of PCR, molecular diagnostic approaches have been revolutionized. Specific PCR amplification of nucleic acid sequences allows easy override of Low-level Detection (LOD) by fluorescence detection. In this reaction, specific primers and a DNA thermo-stable polymerase enzyme are required, along with nucleotides and a thermo-cycler that regulates the temperature strictly cyclically. Figure 3 depicting PCR-based biosensors used for the detection and diagnosis of nucleic acids. This figure showcases the integration of PCR techniques with advanced biosensors, emphasizing their enhanced sensitivity, specificity, and rapid detection capabilities in clinical diagnostics. Based on the desired information, PCR could be coupled to various techniques in the analysis workflow. Real-time PCR can detect pathogenic microbes rapidly and quantitatively using DNA microarrays, gel electrophoresis and electrophoresis gels for identifying and visualizing genetic material.39
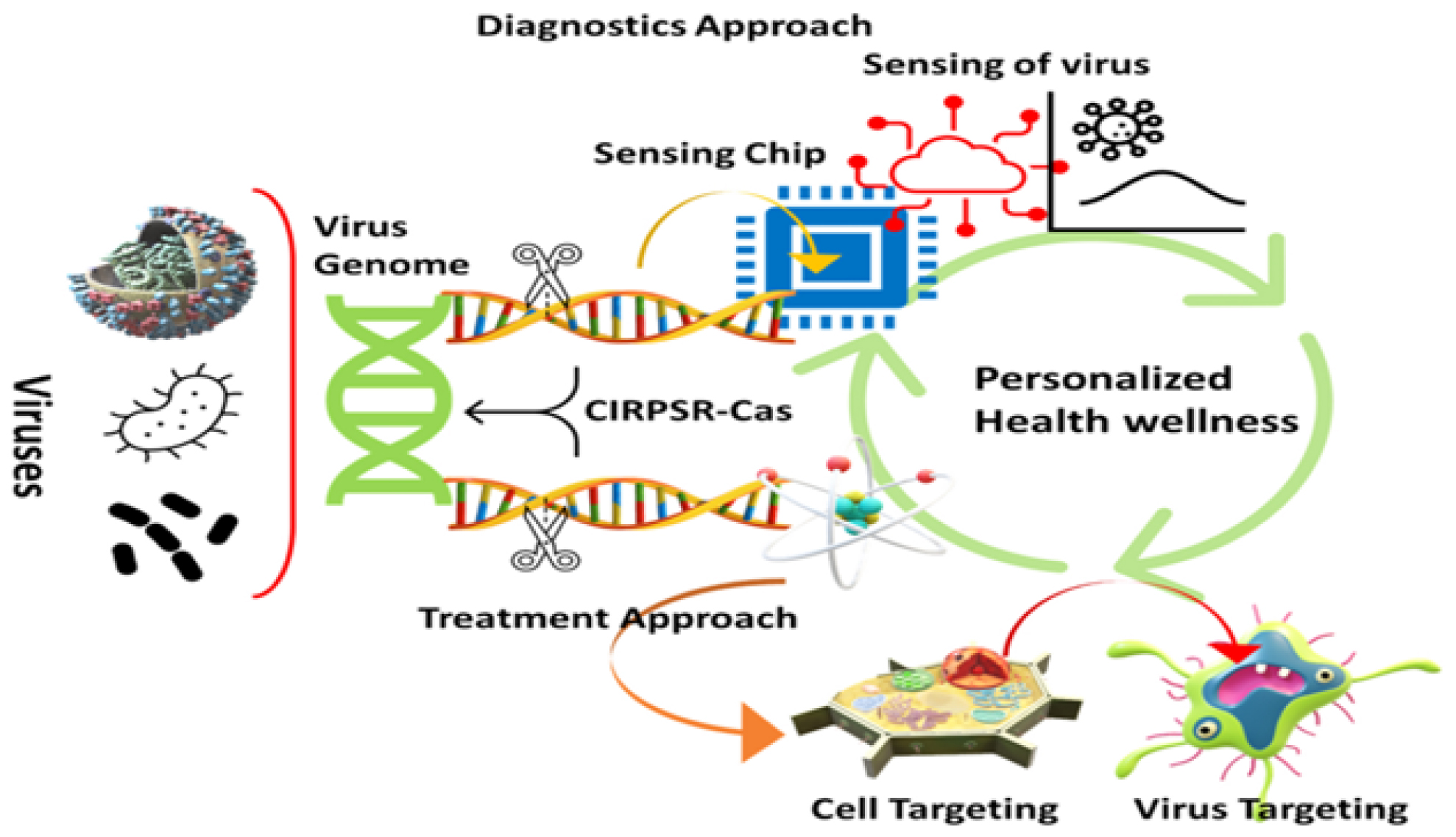
Figure 1:
CRISPR-Cas-enabled nanotechnology for effective disease diagnosis and treatment.
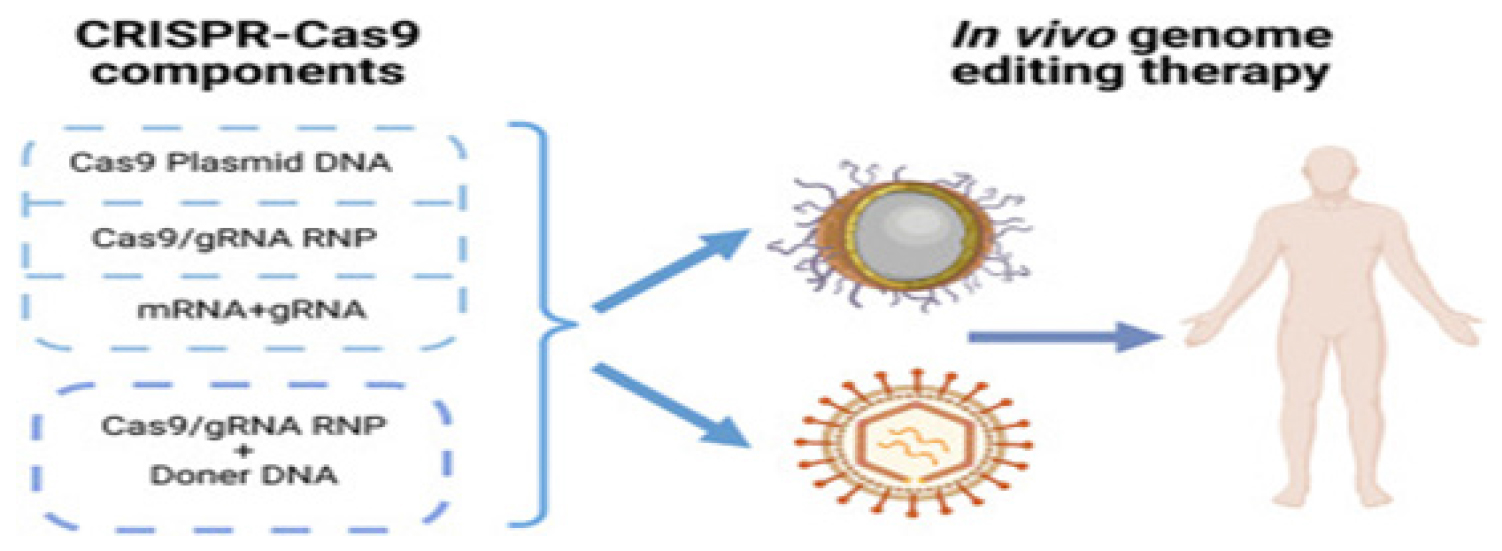
Figure 2:
Challenges and progress in CRISPR-Cas therapy.
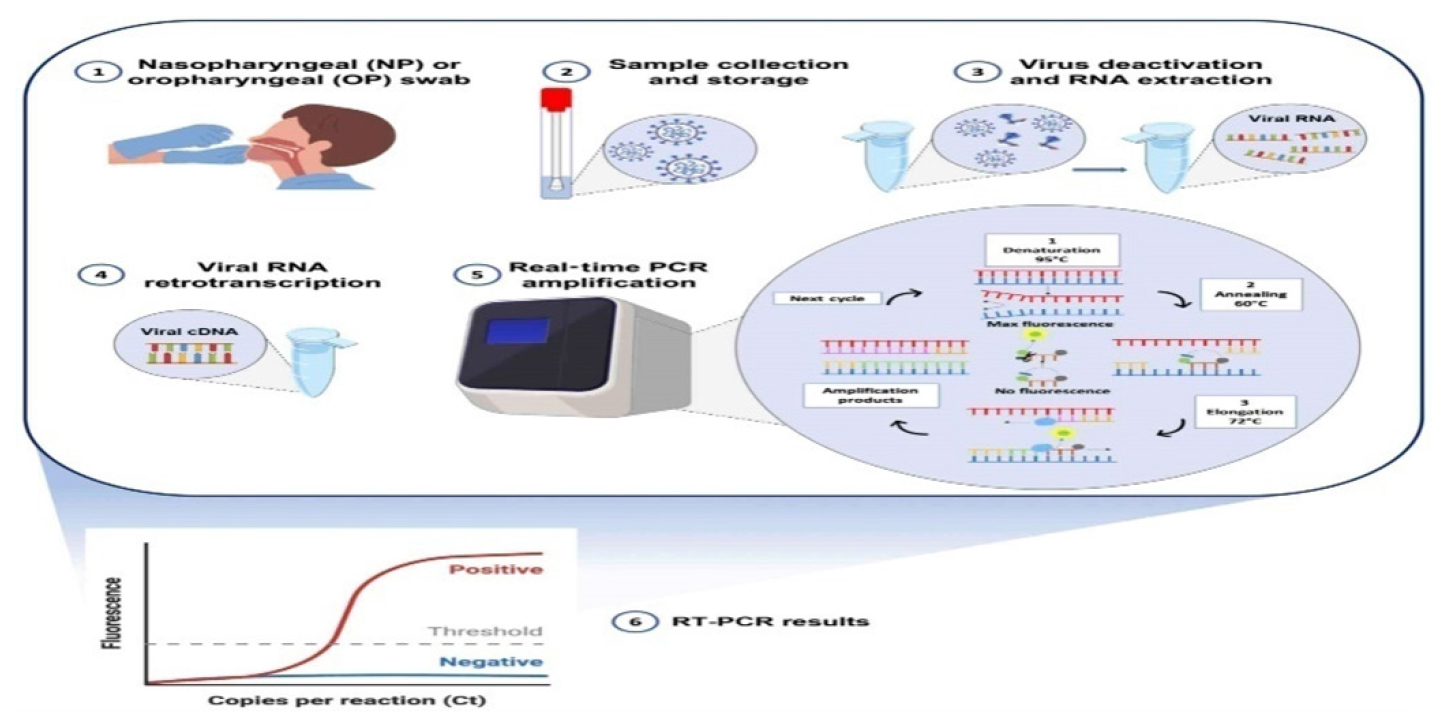
Figure 3:
PCR-based biosensors for detection and diagnosis of nucleic acids.
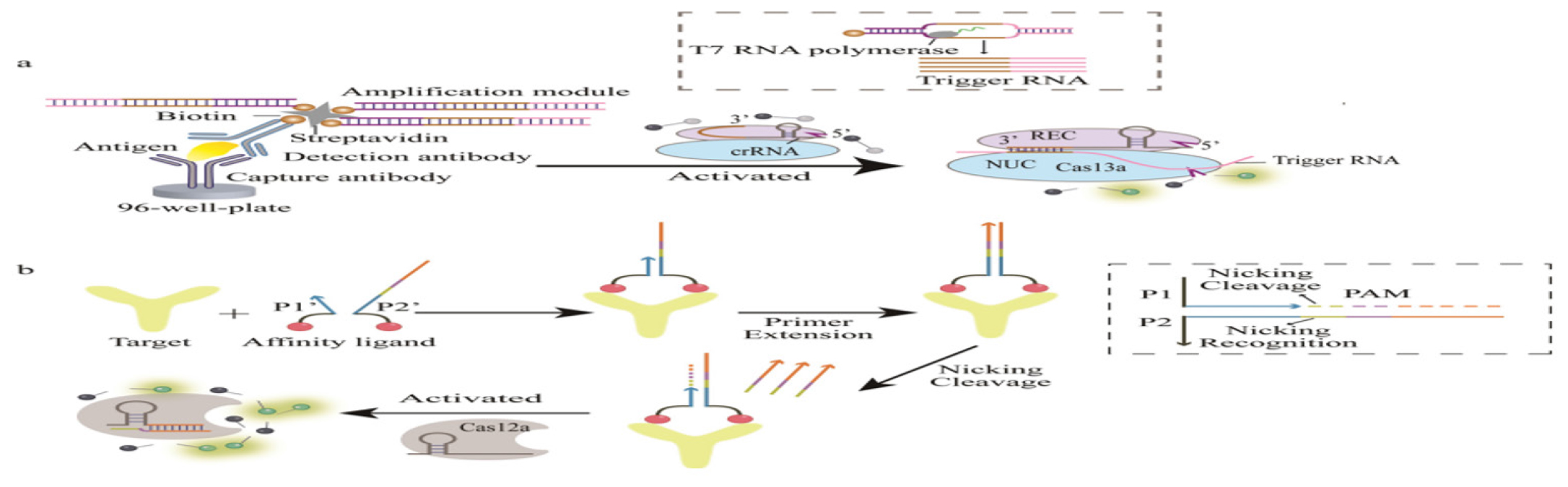
Figure 4:
Schematic diagram of signal amplification (Immunosobent detection).
Cas effector (Type-II model)
Many gene editing applications use the Cas9 enzyme, the most popular type-II enzyme, but recent studies have demonstrated that it was also a reliable bio-recognition enzyme.40,41 A table summarizing the most recent papers concerning the development of portable biosensing systems and biosensors as well as the implementation of existing molecular laboratory techniques. Most of the applications used the wild-type Cas9 enzyme (Cas9) or gene-deactivated Cas9 enzyme. Cas9 recognizes and cuts the DNA target in a single turnover activity, as shown in section 2.42 With recent advancements in molecular laboratory techniques, amplification-based methods have become more accurate and effective. In order to replace expensive conventional PCR protocols, innovative strategies employ isothermal amplification techniques in conjunction with the Cas9/sgRNA complex. An Exponential Amplification Reaction (EXPAR) combined with Cas9/sgRNA specific cutting activity has been developed as a method for nucleic acid detection. SYBR green I dye is used in a fluorescent laboratory instrument to measure real-time fluorescent signals.
EXPAR challenges one method of amplifying long singlestranded DNA (ssDNA) with exogenous oligonucleotide primers. It often produces false-positive signals due to primers that amplification targets aren’t dependent on, making EXPAR unsuitable for detection applications. It is, however, possible to overcome this limitation by using the Cas9/sgRNA complex. As Cas9/sgRNA recognizes and cuts ssDNA targets, its products serve as internal primers for amplification. Previously, EXPAR amplification had been applied primarily to mRNA, long ssDNA, or RNA detection. This alternative approach has enabled rapid, efficient and accurate EXPAR amplification.43 NASBACC (NASBA-CRISPR) was an innovative method of using the Cas9 enzyme for the detection of Zika virus. A specially engineered oligonucleotide sensor, called a “toehold switch sensor,” is incorporated into this system to detect Zika virus. Inducing LacZ translation results in the production of chlorophenol red, a purple product (chlorophenol red) from yellow substrates. An amplification strategy based on nucleic acid sequences (NASBA) was used to amplify the Zika virus target. Using the oligonucleotide sensor and the Cas9 enzyme, subsequent target detection was made possible. Its efficiency in detecting viral infections is demonstrated by its ability to detect and distinguish between Zika virus genotypes with single-base resolution in the NASBACC system.44
A liquid-gate graphene Field-Effect Transistor (gFET) was used in the CRISPR-Chip biosensor to enhance the programmability of dCas9/sgRNA specificity. A non-covalent interaction, such as aromatic stacking, attaches dCas9 enzyme to graphene surface through 1-Pyrenebutanoic Acid (PBA). Graphene channels change in current when Cas9/sgRNA interacts with the target gene.
Two distinct mutations associated with Duchenne Muscular Dystrophy (DMD) were detected using this innovative biosensor, but it could be used to analyze any nucleic acid target gene. Several applications can be addressed using CRISPR/Cas9 technology on the CRISPR-Chip.45 Table 1 explores the diverse methods and innovations involving Type II Cas effectors, highlighting their integration into advanced molecular diagnostics and on-site detection technologies.
Name | Cas enzyme | Target | Amplification | Sample | Year | References |
---|---|---|---|---|---|---|
CAS-EXPAR | Cas9 | ssDNA | EXPAR | Buffer contains target | 2018 | 46 |
DNA-FISH | dCas9 | S. aureus | – | Genetic DNA/ Cell lysate | 2017 | 47 |
CRISPR-CHIP | dCas9 | DMAS | – | Buffer | 2019 | 48 |
CRISP-Cas9-SDA-RCA | Cas9 | E. coli | SDA+RCA | Spike spring water | 2020 | 49 |
Type II Cas effectors exploring the various techniques regarding molecular laboratory techniques, portable biosensing systems.
Anti-body assisted Protein detection by CRISPR/Cas
Molecular recognition molecules, antibodies, have two Heavy (H) and Light (L) chains, which were bonded by Non-covalent as well as R-S-S’-R bonds. Molecular determinants in these molecules have the unique ability to recognize and bind with exceptional affinity and specificity to antigen determinants on target proteins.
As biological recognition elements, antibodies play a crucial role in biosensors, especially in immunosensors. Biosensors were the most predominant and essential biosensing platforms for protein detection. Figure 4 demonstrates the mechanisms by which signal amplification enhances the sensitivity and accuracy of immunoassays, crucial for detecting low-abundance biomarkers in diagnostic applications. Immunosensors primarily utilize sandwich assays to detect antibody-antigen interactions. Table 2 presents various CRISPR/Cas approaches that incorporate antibodies for protein detection, comparing their efficacy, detection ranges, and application scenarios in diagnostic assays. The use of proximity CRISPR/Cas12a assays in combination with antigen-antibody recognition was also a promising mode of protein detection.
Aptamer-protein detection
Due to their functional similarity to antibodies, aptamers were often called “chemical antibodies” since they are synthetic oligonucleotide sequences composed of DNA or RNA. The nucleic acid sequences of these molecules, as well as their unique three-dimensional structures, allow them to bind to target molecules with extreme affinities and specificities.52,53 Table 3 details the different CRISPR/Cas biosensor configurations that utilize aptamers for protein detection, including performance metrics such as sensitivity, specificity, and detection limits. Compared to antibodies, aptamers have a number of advantages, such as low immunogenicity, affordability in preparation, long-term storage stability, flexibility in modification, high structural stability, resistance to temperature variations, compact size, consistency across batches, compatibility with nucleic acid signal amplification techniques and versatility in targeting a wide range of molecules.54,55 Figure 5 highlights the process by which CRISPR/Cas systems, coupled with nicking enzymes, enable visual detection of target sequences through colorimetric changes, offering a simple yet effective method for rapid diagnostics. Table 4 highlights the sensitivity, specificity, and overall performance of different sensor designs, providing insights into their effectiveness for direct protein analysis in clinical and research settings.
Method | Target | Signal | References |
---|---|---|---|
AID-Cas | CD63 Positive EVs | Fluorescence | 56 |
Apta-HCR- CRISPR | TEV | Fluorescence | 57 |
Non-enzymatic HCR Powered CRISPR/Cas12a | PSA | Colorimetric | 58 |
Aptamer assisted protein detection by CRISPR/Cas biosensor.
Method | Target | Signal | References |
---|---|---|---|
Nano-CLISA | CEA and PSA | Fluorescence | 59 |
CAFI | Cytokine IFN-W | Fluorescence | 60 |
DNA walker amplified CRISPR/Cas12a | CEA | Fluorescence | 61 |
Dual aptamer-assisted CRISPR/Cas12a | SARS-CoV-2-antigen | Fluorescence | 62 |
Comparative detection aptamer-based sensor without amplification for protein detection.
Other types of protein detection
The CRISPR/Cas system has become a powerful tool for imaging live cells, originally only useful for imaging genomic loci and mRNA.63,64 Contrary to CRISPR/Cas12a, which recognizes dsDNA via PAM sequences, the detection spectrum of targets is greatly limited by CRISPR/Cas12a.65 Analytes could be added to DNA substrates through the design of universal response mechanisms. As a result of the Cas12a/gRNA complex’s ability to recognize unwound DNA substrates without PAM restrictions.66,67 In order to image live cells, the biosensor uses a PAM-free dsDNA substrate generated from recognizing and converting the corresponding pcDNA, which initiates Cas12a nuclease activity by cleaving fluorescent ssDNA signal probes surrounding it. The innovative strategy has been demonstrated to be effective for the detection of several biomolecules, including Adenosine Triphosphate (ATP), microRNAs (miRNAs) and telomerase. Additionally, it can detect enzymes, mRNAs and small molecules inside cells. However, due to the complexity of cytoplasm, the sensitivity and reproducibility of this biosensor were reduced by its reliance on effective collisions. Encapsulating the sensing system components might be possible with DNA technology or liquid-liquid phase separation. This enzyme cleaves double strand from 30 to 50 to produce single strand with a protruding 30 end when it recognizes flat-ended dsDNA.68 The Cas12a biosensor based on ExoIII was used to identify factors (transcription factors) in double strands DNA activator that contain structural repeats of TFs, preventing ExoIII from degrading the double strands.69 A thermal inactivation takes place at 65°C, which activates Cas12a’s trans cleavage activity. NF-kB p50 subunit can be detected using this approach with a Limit of Detection (LOD) of 0.2 pM. TF inhibitors can be screened using this method and their biological activities assessed. Despite the method’s significant limitations, it must be acknowledged that it suffers from prolonged detection times and requires temperature control. Furthermore, cellular nuclear protein extracts may affect the results of the assay.
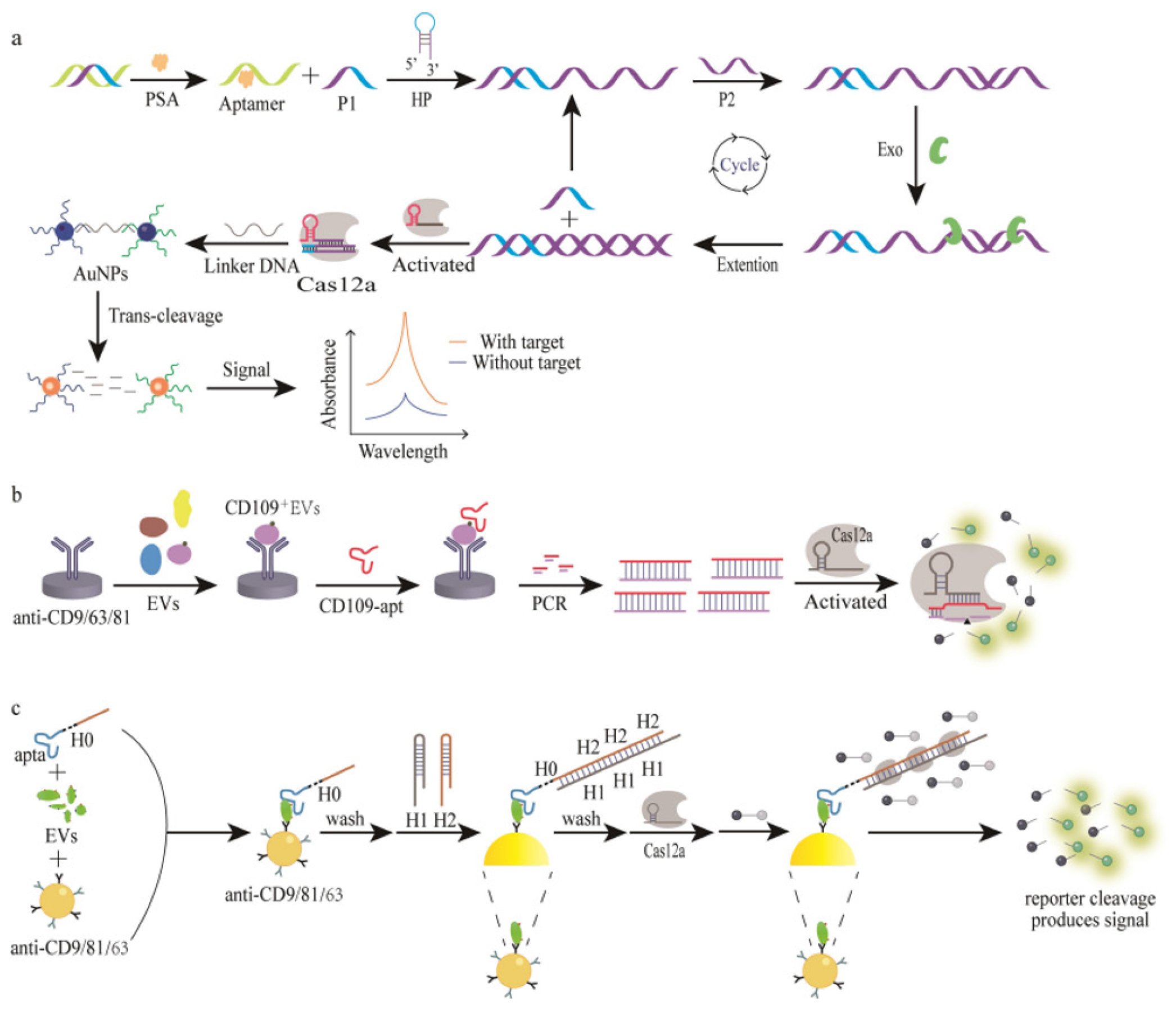
Figure 5:
CRISPR/Cas Colorimetric detection (Nicking-enzyme).
Additionally, intermolecular interactions can also be detected using the CRISPR/Cas system. By utilizing the CRISPR/Cas system, Kim et al developed a rapid method for detecting protein/ small molecule interactions. The activator single-stranded DNA (AD) in this method is modified with a small molecule in order to interact with the target protein. The small molecule interferes with AD’s binding to crRNA, reducing Cas12a’s trans cleavage activity and decreasing fluorescence when it binds to the protein. As a result, Limits of Detection (LOD) were achieved of 0.03 nM for streptavidin/biotin and 0.09 nM for antidigoxin/digoxin.70 This strategy may be used for fast detection of other protein-small molecule interactions, offering new possibilities for analyzing protein-small molecule interactions and finding modulators that are associated with them.
Nanoformulations based drug delivery approaches
The toxicity of conventional viral delivery systems based on retroviruses and adenoviruses prevents their widespread use, including initiation of immune system responses and invasion into recipient chromosomes.71 Despite their limited packing abilities, adeno-associated viruses were not able to effectively deliver CRISPR/Cas9 components. The distribution performance of non-viral delivery systems, however, was poor, compared with genome-editing components.72 Nanotechnology has enabled the development of non-viral genome editing mechanisms
Several techniques have been developed to enhance delivery effectiveness, including endosomal escape, active targeting and stimulus-responsive cargo release. Researchers had delivered CRISPR/Cas9 to cells using natural extracellular vesicles and synthetic nanoparticles
Lipid based delivery
During late endosome formation, Lipid Nanoparticles (LNPs) enhanced with ionizable cationic lipids acquires a positive charge so that they can be released from the endosome. In this study, lipid nanocarriers were tested for their ability to deliver CRISPR/Cas9. Polo-like kinase-1 (Plk1), a mitosis regulator often overexpressed in cancer cells, was targeted by lipid-gold nanoparticles in one study. The researchers complexed HIV-1 Tat peptide cationic gold nanoclusters electrostatically with Cas9 protein and Plk1-targeting plasmid DNA using single-guide RNA (sgRNA) to enhance nuclear targeting. Using liver-targeting LNPs, CRISPR-Cas9 was delivered by CRISPR-Cas9 in a preclinical mouse model of transthyretin amyloidosis to downregulate the target gene (ATTR) and reduce serum levels of dysfunctional transthyretin protein by 70-80%.73,74
A lecithin-based liposomal Nanocarrier particle (NL) delivered Cas9-RNPs to target the DPP-4 gene in Type 2 Diabetes Mellitus. NL particles based on lecithin are readily absorbed by hepatocytes, indicating that prolonged NL accumulation in the liver might increase gene disruption potency. By downregulating DPP-4 through NL Cas9-RNP treatment, glucose tolerance and insulin sensitivity were improved.
Polymeric Peptide Based Delivery
Biological transfection could be accomplished using various polymers to deliver DNA, RNA, or Cas9. A viable approach would be to encapsulate them in polymeric carriers. Polycaprolactone (PCL) or Polylactic/Glycolic Acid copolymers (PLGA) were examples of such carriers. Nanoparticles could be coated with these polymer coatings using natural monomers. In order to achieve well-dispersed aqueous nanoparticles, their hydrophilic nature reduces dipole attractions. Encapsulation and delivery of the Cas enzyme were enhanced by this hydrophilic environment.75
As a result of the polypeptide PEG nanoparticles vector, Plk1 expression levels significantly decreased and tumor cell proliferation decreased, extending rodent survival. Plk1 deletion was 100% effective in HeLa malignant cells.76
In murine macrophages derived from bone marrow, fluorescent-labeled high molecular weight CRISPR/Cas9 plasmid DNA was transfected with PLGA nanoparticles. Nanoprecipitation, which incorporates a hydrogen-based organic polymer and medicinal solution in aqueous solution with a polymer surfactant, was used for this transfection process.77
Cell-fusion type delivery
Hybridized extracellular vesicles were used for the first time to administer CRISPR/Cas9 to MSCs. Exosomes and liposomes were incubated together to create these hybrid nanoparticles. Gene therapy has been transformed by CRISPR/Cas9, which overcomes decades-old challenges. In MSCs, the hybrid nanoparticles were released and the encapsulated genes were transcribed. Hybrid exosome-liposome nanoparticles enable the delivery of CRISPR/ Cas9 mechanisms to MSCs, making them potential in vivo gene manipulation tools.78,79
Novel methodology for efficacy and high retention
Specifically targeting the genome in individual cells, tissues and organs remains a major challenge in gene therapy. Biodegradable, non-toxic, low-cost and efficient delivery vectors should also be desirable.80 Viral vector side effects can be mitigated by carefully selecting the delivery vector. Biomedical studies may be concerned about undesired DNA integration into the host genome with Adeno-Associated Viruses (AAVs) and adenoviruses. A number of advantages are available with nonviral vectors, such as peptides that penetrate cells, lipid nanoparticles, polymers and gold nanoparticles. It reduces off-target effects by scaling up easily, minimizing immunogenicity and delivering the CRISPR/Cas mechanism in Ribonucleoprotein Complexes (RNPs).81
Specific nucleic acid sequences in AAV vector genomes activate Toll-Like Receptor 9 (TLR9), triggering interferon production, which enhances Major Histocompatibility Complex (MHC) expression in immune cells and epithelium. A preexisting Memory T Cell may recognize Cas9 and activate the adaptive immune response. PEGylated lipids stabilize and prolong the life of nanoparticles by facilitating endocytosis and preventing nonspecific interactions. Multi-dose administration of lipid and PEG-DMG incorporated into an LNP-based delivery system resulted in long-term liver editing of approximately 70%.82,83
CONCLUSION
In nucleic acid analysis, CRISPR-based biosensors show promising advancements and their potential for detection of proteins. As a result of the molecular properties of aptamers, CRISPR/ Cas biosensors combining antibodies have improved detection limits and expanded the detection range. It will be crucial to use biomaterials for efficient delivery of CRISPR systems, particularly non-viral vectors designed to pack cargo efficiently. CRISPR/ Cas systems combined with advanced techniques such as bioinformatics, automation and high-throughput screening may be able to accelerate the practical application of biosensors based on CRISPR. By leveraging genetic testing to identify protective variants, these tests could assist in finding genes associated with drug resistance and the development of therapeutics for genetic and other diseases.
Cite this article:
Devaraji M, Ravikumar L. Crispr Revolution: Cutting-Edge Biosensors and Nanomedicine for Precision Health. Int. J. Pharm. Investigation. 2025;15(1):57-66.
ACKNOWLEDGEMENT
The illustrations for the review are prepared using Biorender.com.
References
- Fauci AS. Infectious diseases: considerations for the 21st century. Clin Infect Dis. 2001;32(5):675-85. [PubMed] | [CrossRef] | [Google Scholar]
- Zhao L, Zhang X, Wang X, Guan X, Zhang W, Ma J, et al. Recent advances in selective photothermal therapy of tumor. J Nanobiotechnology. 2021;19(1):335 [PubMed] | [CrossRef] | [Google Scholar]
- Tutty MA, Vella G, Vennemann A, Wiemann M, Prina-Mello A. Evaluating nanobiomaterial-induced DNA strand breaks using the alkaline comet assay. Drug Deliv Transl Res. 2022;12(9):2243-58. Erratum in: Drug Deliv transl res. 2022 Jun 19 [PubMed] | [CrossRef] | [Google Scholar]
- Menon GR, Singh L, Sharma P, Yadav P, Sharma S, Kalaskar S, et al. National Burden Estimates of healthy life lost in India, 2017: an analysis using direct mortality data and indirect disability data. Lancet Glob Health. 2019;7(12) [PubMed] | [CrossRef] | [Google Scholar]
- Han HS, Choi KY. Advances in nanomaterial-mediated photothermal cancer therapies: toward clinical applications. Biomedicines. 2021;9(3):305 [PubMed] | [CrossRef] | [Google Scholar]
- Bakhrebah MA, Nassar MS, Alsuabeyl MS, Zaher WA, Meo SA. CRISPR technology: new paradigm to target the infectious disease pathogens. Eur Rev Med Pharmacol Sci. 2018;22(11):3448-52. [PubMed] | [CrossRef] | [Google Scholar]
- Walker DH. Principles of diagnosis of infectious diseases. Pathobiol Hum Dis. 2014:222-5. [CrossRef] | [Google Scholar]
- Watzinger F, Ebner K, Lion T. Detection and monitoring of virus infections by real-time PCR. Mol Aspects Med. 2006;27(2-3):254-98. [PubMed] | [CrossRef] | [Google Scholar]
- Foss DV, Hochstrasser ML, Wilson RC. Clinical applications of CRISPR-based genome editing and diagnostics. Transfusion. 2019;59(4):1389-99. [PubMed] | [CrossRef] | [Google Scholar]
- Verma R, Sahu R, Singh DD, Egbo TE, CRISPR A. A CRISPR/Cas9 based polymeric nanoparticles to treat/inhibit microbial infections. Semin Cell Dev Biol. 2019;96:44-52. [PubMed] | [CrossRef] | [Google Scholar]
- Rocha LF, Braga LA, Mota FB. Gene editing for treatment and prevention of human diseases: A global survey of gene editing-related researchers. Hum Gene Ther. 2020;31(15-16):852-62. [PubMed] | [CrossRef] | [Google Scholar]
- Torres-Ruiz R, Rodriguez-Perales S. CRISPR-Cas9 technology: applications and human disease modelling. Brief Funct Genomics. 2017;16(1):4-12. [PubMed] | [CrossRef] | [Google Scholar]
- Trevisan M, Palù G, Barzon L. Genome editing technologies to fight infectious diseases. Expert Rev Anti-Infect Ther. 2017;15(11):1001-13. [PubMed] | [CrossRef] | [Google Scholar]
- Strich JR, Chertow DS. CRISPR-Cas biology and its application to infectious diseases. J Clin Microbiol. 2019;57(4):e01307-18. [PubMed] | [CrossRef] | [Google Scholar]
- Mustafa MI, Makhawi AM. Sherlock and DETECTR: CRISPR-Cas systems as potential rapid diagnostic tools for emerging infectious diseases. J Clin Microbiol. Array;Array(Array):Array-20. Retraction in: J Clin Microbiol [PubMed] | [CrossRef] | [Google Scholar]
- Yadav N, Narang J, Chhillar AK, Rana JS. CRISPR: A new paradigm of theranostics. Nanomedicine. 2021;33:102350 [PubMed] | [CrossRef] | [Google Scholar]
- Hillaireau H, Couvreur P. Nanocarriers’ entry into the cell: relevance to drug delivery. Cell Mol Life Sci. 2009;66(17):2873-96. [PubMed] | [CrossRef] | [Google Scholar]
- Kumar P, Malik YS, Ganesh B, Rahangdale S, Saurabh S, Natesan S, et al. CRISPR-Cas system: an approach with potentials for COVID-19 diagnosis and therapeutics. Front Cell Infect Microbiol. 2020;10:576875 [PubMed] | [CrossRef] | [Google Scholar]
- Lone BA, Karna SK, Ahmad F, Shahi N, Pokharel YR. CRISPR/Cas9 system: A bacterial tailor for genomic engineering. Genet Res Int. 2018;2018:3797214 [PubMed] | [CrossRef] | [Google Scholar]
- Rodríguez-Rodríguez DR, Ramírez-Solís R, Garza-Elizondo MA, Garza-Rodríguez ML, Barrera-Saldaña HA. Genome editing: A perspective on the application of CRISPR/ Cas9 to study human diseases (Review) [review]. Int J Mol Med. 2019;43(4):1559-74. [PubMed] | [CrossRef] | [Google Scholar]
- Jiang F, Doudna JA. CRISPR-Cas9 structures and mechanisms. Annu Rev Biophys. 2017;46:505-29. [PubMed] | [CrossRef] | [Google Scholar]
- Kennedy EM, Cullen BR. Gene Editing: A new tool for viral disease. Annu Rev Med. 2017;68:401-11. [PubMed] | [CrossRef] | [Google Scholar]
- Barrangou R, Fremaux C, Deveau H, Richards M, Boyaval P, Moineau S, et al. CRISPR provides acquired resistance against viruses in prokaryotes. Science. 2007;315(5819):1709-12. [PubMed] | [CrossRef] | [Google Scholar]
- Strich JR, Chertow DS. CRISPR-Cas biology and its application to infectious diseases. J Clin Microbiol. 2019;57(4):01307-18. [PubMed] | [CrossRef] | [Google Scholar]
- Rath D, Amlinger L, Rath A, Lundgren M. The CRISPR-Cas immune system: biology, mechanisms and applications. Biochimie. 2015;117:119-28. [PubMed] | [CrossRef] | [Google Scholar]
- Jolany Vangah S, Katalani C, Booneh HA, Hajizade A, Sijercic A, Ahmadian G, et al. CRISPR-based diagnosis of infectious and noninfectious diseases. Biol Proced Online. Array;Array(1):Array Erratum in: Biol Proced Online [PubMed] | [CrossRef] | [Google Scholar]
- Hille F, Charpentier E. CRISPR-Cas: biology, mechanisms and relevance. Philos Trans R Soc Lond B Biol Sci. 2016;371(1707):20150496 [PubMed] | [CrossRef] | [Google Scholar]
- Carte J, Wang R, Li H, Terns RM, Terns MP. Cas6 is an endoribonuclease that generates guide RNAs for invader defense in prokaryotes. Genes Dev. 2008;22(24):3489-96. [PubMed] | [CrossRef] | [Google Scholar]
- Pougach K, Semenova E, Bogdanova E, Datsenko KA, Djordjevic M, Wanner BL, et al. Transcription, processing and function of CRISPR cassettes in Escherichia coli. Mol Microbiol. 2010;77(6):1367-79. [PubMed] | [CrossRef] | [Google Scholar]
- Yadalam PK, Arumuganainar D, Anegundi RV, Shrivastava D, Alftaikhah SA, Almutairi HA, et al. CRISPR-Cas-based adaptive immunity mediates phage resistance in periodontal red complex pathogens. Microorganisms. 2023;11(8):2060 [PubMed] | [CrossRef] | [Google Scholar]
- Nam KH, Haitjema C, Liu X, Ding F, Wang H, DeLisa MP, et al. Cas5d protein processes pre-crRNA and assembles into a cascade-like interference complex in subtype I-C/ Dvulg CRISPR-Cas system. Structure. 2012;20(9):1574-84. [PubMed] | [CrossRef] | [Google Scholar]
- Wiedenheft B, van Duijn E, Bultema JB, Waghmare SP, Zhou K, Barendregt A, et al. RNA-guided complex from a bacterial Immune system enhances target recognition through seed sequence interactions. Proc Natl Acad Sci U S A. Array;Array(Array):Array-7. Erratum in: Proc Natl Acad Sci USA [CrossRef] | [Google Scholar]
- Bultema J, Bultema Jelle B, Waghmare Sakharam, Waghmare Sakharam P, Heck Albert, Heck Albert JR, Boekema Egbert, Boekema Egbert J, Dickman Mark, Dickman Mark J, et al. Erratum in: Proc Natl Acad Sci U S A. 2011;108(36):15010 [PubMed] | [CrossRef] | [Google Scholar]
- Barrangou R. CRISPR-Cas systems and RNA-guided interference. Wiley Interdiscip Rev RNA. 2013;4(3):267-78. [PubMed] | [CrossRef] | [Google Scholar]
- Marraffini LA, Sontheimer EJ. CRISPR interference: RNA-directed adaptive immunity in bacteria and archaea. Nat Rev Genet. 2010;11(3):181-90. [PubMed] | [CrossRef] | [Google Scholar]
- Váradi L, Luo JL, Hibbs DE, Perry JD, Anderson RJ, Orenga S, et al. Methods for the detection and identification of pathogenic bacteria: past, present, and future. Chem Soc Rev. 2017;46(16):4818-32. [PubMed] | [CrossRef] | [Google Scholar]
- Tsalik EL, Bonomo RA, Fowler VG. New molecular diagnostic approaches to bacterial infections and antibacterial resistance. Annu Rev Med. 2018;69:379-94. [PubMed] | [CrossRef] | [Google Scholar]
- Hu L, Ru K, Zhang L, Huang Y, Zhu X, Liu H, et al. Fluorescence in situ hybridization (FISH): an increasingly demanded tool for biomarker research and personalized medicine. Biomark Res. 2014;2(1):3 [PubMed] | [CrossRef] | [Google Scholar]
- Watzinger F, Ebner K, Lion T. Detection and monitoring of virus infections by real-time PCR. Mol Aspects Med. 2006;27(2-3):254-98. [PubMed] | [CrossRef] | [Google Scholar]
- Zoppo M, Luca MD, Villarreal SN, Poma N, Barrasa MI, Bottai D, et al. A CRISPR/ Cas9-based strategy to simultaneously inactivate the entire ALS gene family in Candida orthopsilosis. Future Microbiol. 2019;14:1383-96. [PubMed] | [CrossRef] | [Google Scholar]
- Zoppo M, Di Luca M, Franco M, Rizzato C, Lupetti A, Stringaro A, et al. CpALS4770 and CpALS4780 contribution to the virulence of
. Microbiol Res. 2020;231:126351 [PubMed] | [CrossRef] | [Google Scholar] - Koo B, Kim DE, Kweon J, Jin CE, Kim SH, Kim Y, et al. CRISPR/dCas9-mediated biosensor for detection of tick-borne diseases. Sens Actuators B Chem. 2018;273:316-21. [PubMed] | [CrossRef] | [Google Scholar]
- Huang M, Zhou X, Wang H, Xing D. Clustered regularly interspaced short palindromic repeats/Cas9 triggered isothermal amplification for site-specific nucleic acid detection. Anal Chem. 2018;90(3):2193-200. [PubMed] | [CrossRef] | [Google Scholar]
- Pardee K, Green AA, Takahashi MK, Braff D, Lambert G, Lee JW, et al. Rapid, low-cost detection of Zika virus using programmable biomolecular components. Cell. 2016;165(5):1255-66. [PubMed] | [CrossRef] | [Google Scholar]
- Hajian R, Balderston S, Tran T, deBoer T, Etienne J, Sandhu M, et al. Detection of unamplified target genes via CRISPR-Cas9 immobilized on a graphene field-effect transistor. Nat Biomed Eng. 2019;3(6):427-37. [PubMed] | [CrossRef] | [Google Scholar]
- Huang M, Zhou X, Wang H, Xing D. Clustered regularly interspaced short palindromic repeats/Cas9 triggered isothermal amplification for site-specific nucleic acid detection. Anal Chem. 2018;90(3):2193-200. [PubMed] | [CrossRef] | [Google Scholar]
- Guk K, Keem JO, Hwang SG, Kim H, Kang T, Lim EK, et al. A facile, rapid and sensitive detection of MRSA using a CRISPR-mediated DNA FISH method, antibody-like dCas9/ sgRNA complex. Biosens Bioelectron. 2017;95:67-71. [PubMed] | [CrossRef] | [Google Scholar]
- Hajian R, Balderston S, Tran T, deBoer T, Etienne J, Sandhu M, et al. Detection of unamplified target genes via CRISPR-Cas9 immobilized on a graphene field-effect transistor. Nat Biomed Eng. 2019;3(6):427-37. [PubMed] | [CrossRef] | [Google Scholar]
- Sun X, Wang Y, Zhang L, Liu S, Zhang M, Wang J, et al. CRISPR-Cas9 triggered two-step isothermal amplification method for E. coli O157:H7 detection based on a metal– organic framework platform. Anal Chem. 2020;92(4):3032-41. [PubMed] | [CrossRef] | [Google Scholar]
- Li Y, Mansour H, Watson CJ, Tang Y, MacNeil AJ, Li F, et al. Amplified detection of nucleic acids and proteins using an isothermal proximity CRISPR Cas12a assay. Chem Sci. 2021;12(6):2133-7. [PubMed] | [CrossRef] | [Google Scholar]
- Chen Q, Tian T, Xiong E, Wang P, Zhou X. CRISPR/Cas13a signal amplification linked immunosorbent assay for femtomolar protein detection. Anal Chem. 2020;92(1):573-7. [PubMed] | [CrossRef] | [Google Scholar]
- Wu L, Wang Y, Xu X, Liu Y, Lin B, Zhang M, et al. Aptamer-based detection of circulating targets for precision medicine. Chem Rev. 2021;121(19):12035-105. [PubMed] | [CrossRef] | [Google Scholar]
- Yu H, Alkhamis O, Canoura J, Liu Y, Xiao Y. Advances and challenges in small-molecule DNA aptamer isolation, characterization and sensor development. Angew Chem Int Ed Engl. 2021;60(31):16800-23. [PubMed] | [CrossRef] | [Google Scholar]
- Röthlisberger P, Hollenstein M. Aptamer chemistry. Adv Drug Deliv Rev. 2018;134:3-21. [PubMed] | [CrossRef] | [Google Scholar]
- Wang T, Chen C, Larcher LM, Barrero RA, Veedu RN. Three decades of nucleic acid aptamer technologies: lessons learned, progress and opportunities on aptamer development. Biotechnol Adv. 2019;37(1):28-50. [PubMed] | [CrossRef] | [Google Scholar]
- Zhao X, Zeng L, Mei Q, Luo Y. Allosteric probe-initiated wash-free method for sensitive extracellular vesicle detection through dual cycle-assisted CRISPR-Cas12a. ACS Sens. 2020;5(7):2239-46. [PubMed] | [CrossRef] | [Google Scholar]
- Xing S, Lu Z, Huang Q, Li H, Wang Y, Lai Y, et al. An ultrasensitive hybridization chain reaction-amplified CRISPR-Cas12a aptasensor for extracellular vesicle surface protein quantification. Theranostics. 2020;10(22):10262-73. [PubMed] | [CrossRef] | [Google Scholar]
- Wang W, Liu J, Wu LA, Ko CN, Wang X, Lin C, et al. Nicking enzyme-free strand displacement amplification-assisted CRISPR-Cas-based colorimetric detection of prostate-specific antigen in serum samples. Anal Chim Acta. 2022;1195:339479 [PubMed] | [CrossRef] | [Google Scholar]
- Zhao Q, Pan Y, Luan X, Gao Y, Zhao X, Liu Y, et al. Nano-immunosorbent assay based on Cas12a/crRNA for ultra-sensitive protein detection. Biosens Bioelectron. 2021;190:113450 [PubMed] | [CrossRef] | [Google Scholar]
- Deng F, Li Y, Qiao L, Goldys E. A CRISPR/Cas12a-assisted on-fibre immunosensor for ultrasensitive small protein detection in complex biological samples. Anal Chim Acta. 2022;1192:339351 [PubMed] | [CrossRef] | [Google Scholar]
- Li CY, Liu JX, Liu YH, Gao JL, Zheng B, Liu D, et al. An exceptional and universal DNA walker amplified “one-to-many” CRISPR/Cas12a-mediated fluorescent biosensor for ultrasensitive detection of non-DNA biomarkers. Sens Actuators B. 2022;361:131743 [CrossRef] | [Google Scholar]
- Zhao X, Wang Z, Yang B, Li Z, Tong Y, Bi Y, et al. Integrating PCR-free amplification and synergistic sensing for ultrasensitive and rapid CRISPR/Cas12a-based SARS-CoV-2 antigen detection. Synth Syst Biotechnol. 2021;6(4):283-91. [PubMed] | [CrossRef] | [Google Scholar]
- Sun NH, Chen DY, Ye LP, Sheng G, Gong JJ, Chen BH, et al. CRISPR-Sunspot: imaging of endogenous low-abundance RNA at the single-molecule level in live cells. Theranostics. 2020;10(24):10993-1012. [PubMed] | [CrossRef] | [Google Scholar]
- Mao S, Ying Y, Wu X, Krueger CJ, Chen AK. CRISPR/dual-FRET molecular beacon for sensitive live-cell imaging of non-repetitive genomic loci. Nucleic Acids Res. 2019;47(20):131 [PubMed] | [CrossRef] | [Google Scholar]
- Karvelis T, Bigelyte G, Young JK, Hou Z, Zedaveinyte R, Budre K, et al. PAM recognition by miniature CRISPR-Cas12f nucleases triggers programmable double-stranded DNA target cleavage. Nucleic Acids Res. 2020;48(9):5016-23. [PubMed] | [CrossRef] | [Google Scholar]
- Jeon Y, Choi YH, Jang Y, Yu J, Goo J, Lee G, et al. Direct observation of DNA target searching and cleavage by CRISPR-Cas12a. Nat Commun. 2018;9(1):2777 [PubMed] | [CrossRef] | [Google Scholar]
- Mol CD, Kuo CF, Thayer MM, Cunningham RP, Tainer JA. Structure and function of the multifunctional DNA-repair enzyme exonuclease III. Nature. 1995;374(6520):381-6. [PubMed] | [CrossRef] | [Google Scholar]
- Selvakumar SC, Preethi KA, Ross K, Tusubira D, Khan MW, Mani P, et al. CRISPR/Cas9 and next generation sequencing in the personalized treatment of Cancer. Mol Cancer. 2022;21(1):83 [PubMed] | [CrossRef] | [Google Scholar]
- Kim H, Lee S, Yoon J, Song J, Park HG. CRISPR/Cas12a collateral cleavage activity for simple and rapid detection of protein/small molecule interaction. Biosens Bioelectron. 2021;194:113587 [PubMed] | [CrossRef] | [Google Scholar]
- Kim D, Le QV, Wu Y, Park J, Oh YK. Nanovesicle-mediated delivery systems for CRISPR/ Cas genome editing. Pharmaceutics. 2020;12(12):1233 [PubMed] | [CrossRef] | [Google Scholar]
- Wu Z, Yang H, Colosi P. Effect of genome size on AAV vector packaging. Mol Ther. 2010;18(1):80-6. [PubMed] | [CrossRef] | [Google Scholar]
- Sheridan C. CRISPR therapeutics push into human testing. Nat Biotechnol. 2017;35(1):3-5. [PubMed] | [CrossRef] | [Google Scholar]
- Norgren N, Olsson M, Nyström H, Ericzon BG, de Tayrac M, Genin E, et al. Gene expression profile in hereditary transthyretin amyloidosis: differences in targeted and source organs. Amyloid. 2014;21(2):113-9. [PubMed] | [CrossRef] | [Google Scholar]
- Kumar R, Mondal K, Panda PK, Kaushik A, Abolhassani R, Ahuja R, et al. Core-shell nanostructures: perspectives towards drug delivery applications. J Mater Chem B. 2020 [PubMed] | [CrossRef] | [Google Scholar]
- Wang HX, Song Z, Lao YH, Xu X, Gong J, Cheng D, et al. Nonviral gene editing via CRISPR/Cas9 delivery by membrane-disruptive and endosomolytic helical polypeptide. Proc Natl Acad Sci U S A. 2018;115(19):4903-8. [PubMed] | [CrossRef] | [Google Scholar]
- Paramasivam G, Sanmugam A, Palem VV, Sevanan M, Sairam AB, Nachiappan N, et al. Nanomaterials for detection of biomolecules and delivering therapeutic agents in theragnosis: a review. Int J Biol Macromol. 2024;254(2):127904 [PubMed] | [CrossRef] | [Google Scholar]
- Lin Y, Wu J, Gu W, Huang Y, Tong Z, Huang L, et al. Exosome-liposome hybrid nanoparticles deliver CRISPR/Cas9 system in MSCs. Adv Sci (Weinh). 2018;5(4):1700611 [PubMed] | [CrossRef] | [Google Scholar]
- Chouhan RS, Shah M, Prakashan D, P R R, Kolhe P, Gandhi S, et al. Emerging trends and recent progress of MXene as a promising 2D material for point of care (POC) diagnostics. Diagnostics (Basel). 2023;13(4):697 [PubMed] | [CrossRef] | [Google Scholar]
- Sahel DK, Mittal A, Chitkara D. CRISPR/Cas system for genome editing: progress and prospects as a therapeutic tool. J Pharmacol Exp Ther. 2019;370(3):725-35. [PubMed] | [CrossRef] | [Google Scholar]
- Wilbie D, Walther J, Mastrobattista E. Delivery aspects of CRISPR/Cas for genome editing. Acc Chem Res. 2019;52(6):1555-64. [PubMed] | [CrossRef] | [Google Scholar]
- Finn JD, Smith AR, Patel MC, Shaw L, Youniss MR, van Heteren J, et al. A single administration of CRISPR/Cas9 lipid nanoparticles achieves robust and persistent genome editing. Cell Rep. 2018;22(9):2227-35. [PubMed] | [CrossRef] | [Google Scholar]
- Tay LS, Palmer N, Panwala R, Chew WL, Mali P. Translating CRISPR-Cas therapeutics: approaches and challenges. CRISPR J. 2020;3(4):253-75. [PubMed] | [CrossRef] | [Google Scholar]
- Mishra S, Webster P, Davis ME. PEGylation significantly affects cellular uptake and intracellular trafficking of non-viral gene delivery particles. Eur J Cell Biol. 2004;83(3):97-111. [PubMed] | [CrossRef] | [Google Scholar]