ABSTRACT
Background
The growing popularity of transdermal patches is attributed to their advantageous characteristics, including the absence of gastric-related problems, prolonged drug release, enhanced safety and stability, and the elimination of the need for punctures during systemic delivery. These features have prompted the exploration of novel techniques and innovations in transdermal delivery systems to optimize drug administration.
Materials and Methods
To address the aforementioned advantages, researchers have focused on incorporating innovative approaches, with a particular emphasis on the utilization of nano-vesicular systems. The nano-vesicular system has gained favour due to its potential to significantly increase drug accumulation at the site of administration, its capacity to easily incorporate a wide range of medications, and its ability to minimize systemic drug absorption. This study undertook an examination of the attempts made in the development and implementation of transdermal nano-vesicular administration.
Results
This study revealed the successful adoption and application of nano-vesicular systems in transdermal drug delivery. The results highlight the efficacy of this approach in enhancing drug accumulation at the administration site and its versatility in accommodating various medications. Additionally, the observed low systemic drug absorption further underscores the potential benefits of transdermal nano-vesicular administration.
Conclusion
In conclusion, the study demonstrates the increasing significance of transdermal patches and the promising role played by nano-vesicular systems in addressing key challenges associated with systemic drug delivery. The successful examination of transdermal nano-vesicular administration by the authors emphasizes the advancements in this field and encourages further exploration of its potential applications in pharmaceutical delivery systems.
INTRODUCTION
The field of Drug Delivery System (DDS) technologies represents a pivotal domain within pharmaceutical research and development, dedicated to refining the intricate processes governing the transport and systemic release of active chemicals. These technologies play a fundamental role in ensuring the targeted and effective delivery of therapeutic agents, with the overarching goal of optimizing their bioavailability and therapeutic impact. This intricate orchestration of drug transport and release has been the subject of extensive scientific inquiry, leading to the development of innovative approaches that hold immense promise in revolutionizing the landscape of medical treatment.1–3 The diversity of drug administration modalities underscores the multifaceted nature of DDS technologies, accommodating different therapeutic needs and patient requirements. Depending on the intended route of delivery, a spectrum of modalities exists, including oral administration, transdermal administration, lung inhalation, mucosal administration, and intravenous injection. Each of these modalities brings its own set of advantages and challenges, allowing for tailored solutions to diverse medical scenarios. This versatility enables healthcare professionals to select the most appropriate delivery method based on factors such as the nature of the drug, the desired pharmacokinetics, and patient-specific considerations.4–6 Among these varied administration modalities, the Transdermal Drug Delivery System (TDDS) emerges as a particularly promising and innovative strategy, especially in the context of chronic ailments. Unlike traditional delivery methods, which may involve invasive procedures or encounter challenges related to gastrointestinal absorption, TDDS offers a non-invasive approach to drug administration through the skin. This feature positions TDDS as an attractive option for long-term treatment regimens, where patient compliance and sustained therapeutic efficacy are paramount. The appeal of TDDS extends beyond its noninvasiveness. By bypassing the digestive tract, TDDS circumvents issues associated with first-pass metabolism, enzymatic degradation, and variability in gastrointestinal absorption. This makes TDDS an appealing choice for medications susceptible to degradation or alterations in the gastrointestinal environment. Additionally, the systemic release of drugs through the skin allows for a controlled and sustained release, providing a valuable tool in the management of chronic conditions where consistent therapeutic levels are crucial.
In essence, the exploration of drug delivery system technologies and the diverse array of administration modalities reflect the dynamic landscape of modern pharmaceutical science. The emphasis on precision, efficacy, and patient-friendly approaches underscores the commitment to advancing therapeutic outcomes. Within this context, the transdermal drug delivery system stands out as a beacon of innovation, holding great promise for the effective and patient-centred management of chronic ailments in the evolving landscape of medical therapeutics.
The advent of Transdermal Drug Delivery Systems (TDDS) has ushered in a paradigm shift in the realm of drug administration, offering a noninvasive alternative that stands as a preferred choice over traditional delivery systems. This preference is rooted in the distinctive advantage of circumventing invasive procedures, providing patients with a more comfortable and convenient means of receiving therapeutic interventions. As a consequence, TDDS has emerged as a pioneering strategy, garnering widespread acclaim for its efficacy in the systemic distribution of therapeutic substances across diverse medical domains.
The impact of TDDS on the distribution of therapeutic substances has been particularly profound, reshaping the landscape of treatment methodologies in key medical areas. Notably, TDDS has played a pivotal role in the management of cardiovascular and central nervous system disorders, hormone therapy, and pain management. The noninvasive nature of TDDS not only addresses patient comfort but also opens avenues for enhanced compliance, especially in cases requiring prolonged or recurrent medication.1,2 Crucially, TDDS offers a distinctive advantage by bypassing the digestive tract, a feature that has far-reaching implications for drug bioavailability and efficacy. The avoidance of the digestive tract eliminates the risk of first-pass metabolism, a phenomenon where drugs undergo significant alteration or degradation before reaching systemic circulation. By evading this process, TDDS ensures that the administered medications maintain their intended pharmacological profiles, contributing to a more predictable and reliable therapeutic outcome.3,4 The benefits of TDDS extend beyond the avoidance of first-pass metabolism. The system allows for drug administration without being hampered by the variable conditions of the digestive environment, including pH fluctuations, enzymatic activities, and the presence of intestinal flora. This not only preserves the integrity of the drug but also mitigates the challenges associated with oral administration, where factors like gastrointestinal pH and enzymatic degradation can impact drug stability and efficacy.
A notable hallmark of TDDS is its remarkable persistence in the pharmaceutical landscape. This enduring appeal can be attributed to the system’s ability to manage medication release under controlled and defined usage limitations. The sustained and controlled release of drugs through the skin enables a continuous therapeutic effect, particularly advantageous in the context of chronic conditions requiring prolonged and consistent treatment.5
The landscape of pharmaceutical advancements has witnessed a transformative stride with the introduction of nano vesicular systems, particularly invasomes, in the realm of transdermal drug delivery. This evolution marks a significant departure from conventional delivery systems, as it enables the inclusion of a diverse spectrum of medicines through the utilization of vesicles encompassing niosomes, liposomes, pro-niosomes, and nanoparticles. This article delves into the exploration of endeavors aimed at advancing nano vesicular Transdermal Drug Delivery Systems (TDDS), shedding light on the remarkable potential and innovations within this cutting-edge domain.
Nanovesicular systems, exemplified by invasomes, have emerged as a forefront strategy for transdermal drug delivery, offering a versatile platform for the incorporation of a wide range of medicinal agents. The utilization of vesicles, ranging from niosomes and liposomes to pro-niosomes and nanoparticles, facilitates targeted and controlled drug delivery. This adaptability is crucial in addressing the diverse therapeutic needs across medical disciplines, ensuring a tailored approach to medication administration.6–8 At the core of this exploration lies the concept of invasomes, a novel family of vesicles that plays a pivotal role in enhancing transdermal medication penetration. The constituents of invasomes include phospholipids, ethanol, and various terpenes or terpene combinations, contributing to their unique structure. Notably, these components exhibit exceptional transdermal penetration characteristics, making invasomes a promising and innovative avenue for overcoming the challenges associated with skin permeation.
Phospholipids form a fundamental component of invasomes, imparting structural integrity to the vesicles. Ethanol, a key constituent, influences the fluidity of the vesicle membrane, enhancing its adaptability for transdermal penetration. The inclusion of different terpenes or terpene combinations further contributes to the permeation-enhancing properties of invasomes. This combination of components creates a synergistic effect, optimizing the ability of invasomes to traverse the skin barrier efficiently.9
The significance of invasomes extends beyond their structural composition, as their distinctive characteristics hold immense potential in improving transdermal drug delivery outcomes. With their remarkable transdermal penetration capabilities, invasomes represent a valuable tool for achieving enhanced drug absorption and bioavailability, potentially revolutionizing the efficacy of transdermal drug delivery systems.10,11
Composition of nanovesicles
The following are the key components of nanovesicles
Within invasomes, the inclusion of specific components such as small amounts of ethanol and various terpenes or terpene mixtures plays a critical role in shaping the characteristics of these vesicles. invasomes, being soft liposomal vesicles, exhibit unique properties that make them well-suited as carriers with enhanced skin penetration capabilities. The composition of invasomes typically includes phospholipids along with a minor amount of alcohol, contributing to their structural integrity and functionality.
Ethanol, present in small quantities, serves multiple functions within invasomes. It influences the fluidity and flexibility of the vesicle membrane, ensuring that invasomes can effectively navigate the skin barrier. This, in turn, enhances the overall skin penetration of the vesicles. Additionally, ethanol can act as a co-solvent, aiding in the solubilization of lipophilic drugs and facilitating their incorporation into the vesicles.12,13 Terpenes, particularly terpenoids like citral, limonene, cineol, and eugenol, are crucial constituents of invasomes. These terpenes contribute to the unique characteristics of invasomes by enhancing their skin penetration capabilities. The presence of terpenoids, in combination with ethanol, further augments the ability of invasomes to act as carriers for therapeutic agents.14,15 The general formula of drugs and terpenes within invasomes is designed to enhance the absorption of both hydrophilic and hydrophobic substances. This versatility is significant in the context of transdermal drug delivery, where a diverse range of drugs with varying solubilities must be efficiently delivered through the skin barrier. Essential oil terpenes, commonly found in invasomes, are frequently utilized as penetration enhancers. This property is particularly advantageous as it aids in overcoming the skin’s natural resistance to the penetration of certain substances, ensuring effective drug delivery.
Importantly, the use of terpenes in invasomes is carefully calibrated to minimize potential skin irritation. Terpenes, when employed sparingly, are known to be less irritating to the skin. The consideration of skin tolerance is vital in designing drug delivery systems, ensuring that the therapeutic benefits are not compromised by adverse reactions or discomfort.15 It is noteworthy that the U.S. Food and Drug Administration (FDA) recognizes terpenes as safe components, reinforcing their acceptance and applicability in pharmaceutical formulations. This acknowledgement adds a layer of assurance regarding the safety profile of invasomes, further supporting their potential as carriers for transdermal drug delivery Figure 1.16
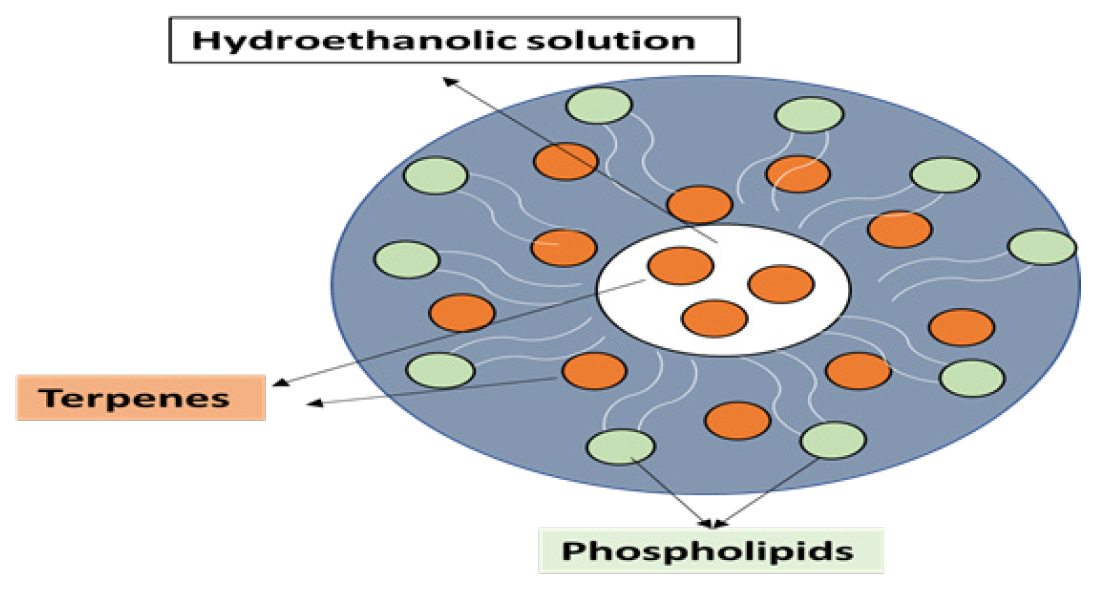
Figure 1:
Invasomes structure.
Phospholipids
The relationship between alcohol and phospholipids within the context of nanovesicles, such as invasomes, is a crucial aspect that influences the structural and functional characteristics of these lipid-based carriers. Phospholipids, which are amphiphilic molecules consisting of hydrophobic acyl chains and a water-loving hydrophilic headgroup, form the fundamental building blocks of these nanovesicles.
The interaction of alcohol with phospholipids plays a key role in determining the properties of the nanovesicles. The hydrophobic acyl chains in phospholipids are capable of associating with alcohol molecules, creating a linkage that influences the overall structure and stability of the vesicles. This interaction is essential for maintaining the integrity of the lipid bilayer, which is crucial for the vesicle’s ability to encapsulate and deliver therapeutic agents.
A remarkable aspect contributing to the versatility of nanovesicles is the diversity of phospholipids that can be utilized in their formulation. The survival and functionality of nanovesicles are attributed to the variations in primary groups, aliphatic chains, and alcohols within different phospholipid classes. This variability in phospholipid sources provides flexibility in tailoring the properties of nanovesicles to meet specific requirements, such as drug compatibility, stability, and skin penetration.
In the context of phospholipid classes, both natural and artificial phospholipids find widespread use in the formulation of nanovesicles. Natural phospholipids sourced from biological membranes offer biocompatibility and are commonly used in various pharmaceutical and cosmetic formulations. Additionally, artificial phospholipids, including PEGylated phospholipids, contribute to the design of nanovesicles with specific characteristics, such as enhanced stability and prolonged circulation time in the body.17 The applications of nanovesicles extend beyond pharmaceutical formulations, finding utility in a variety of compositions, including skincare products. The ability of nanovesicles to encapsulate and deliver active ingredients, such as vitamins or antioxidants, makes them valuable components in cosmetic and dermatological formulations. This versatility highlights the adaptability of nanovesicles for different applications beyond drug delivery.
Furthermore, the procedure for creating nanovesicles often involves the use of hydrogenated phosphatidylcholine. This specific phospholipid plays a crucial role in stabilizing the nanovesicle structure and is commonly employed in the pharmaceutical industry for the production of liposomal and related vesicular drug delivery systems.18
Terpenes
Terpenes, either individually or as mixtures, play a pivotal role in the realm of transdermal drug delivery as penetration enhancers, also known as sorption enhancers or accelerators. These compounds, derived from natural sources such as essential oils, have been extensively studied for their ability to facilitate the permeation of drugs through the skin, effectively lowering the barrier’s resistance. The application of terpenes in very small concentrations has garnered attention in pharmaceutical research due to their potential to enhance the efficacy of transdermal drug delivery systems.
Research on transdermal drug delivery has established that terpenes, when incorporated in minute quantities, can function as penetration enhancers by permeating the skin and reducing the skin barrier’s resistance. The mechanism behind this enhancement involves the modification of the skin’s properties, making it more receptive to the passage of therapeutic agents. Terpenes achieve this by interacting with the lipids in the stratum corneum, the outermost layer of the skin, and disrupting the organization of these lipids. This disruption leads to an increase in the fluidity of the lipid bilayers, allowing for improved diffusion of drugs through the skin.
Terpenes, being classified as Generally Regarded as Safe (GRAS), are deemed suitable for application to the skin due to their minimal risk of causing skin irritation. This recognition by regulatory authorities adds to their appeal as safe and effective components in transdermal drug delivery formulations. The GRAS status implies that, when used in appropriate concentrations, terpenes are unlikely to cause harm or adverse effects on the skin, ensuring their acceptability in pharmaceutical and cosmetic applications.
The use of terpenes as penetration enhancers addresses a significant challenge in transdermal drug delivery, where the skin’s natural barrier properties can limit the absorption of therapeutic agents. By selectively modifying the skin’s structure, terpenes enhance the permeability of the stratum corneum without irritating, making them valuable tools in the development of efficient and patient-friendly transdermal drug delivery systems.19
Ethanol
The introduction of ethanol into nano vesicular systems represents a significant strategy to enhance permeability, influencing various aspects of the system’s properties. The impact of vesicles on nanovascular systems is profound, owing to their unique characteristics such as size, zeta potential, entrapment effectiveness, and skin permeability.
Research findings indicate that the addition of ethanol has notable effects on the size and overall efficacy of vesicles. As ethanol content increases, the effectiveness of vesicles tends to decline. This is attributed to the disintegration of vesicles when ethanol concentration rises beyond a certain threshold. High ethanol concentrations lead to a reduction in the thickness of the vesicle membrane, causing a decrease in the vesicle volume. This phenomenon is associated with the penetration of ethanol into the hydrocarbon chains, altering their net charge and resulting in a decrease in the average size of the vesicles.
The inclusion of ethanol in nanovesicles not only influences their size but also impacts their fluidity. Ethanol can penetrate the tightly packed structure of the Stratum Corneum (SC) lipids, disrupting their arrangement and causing separation. This effect increases the fluidity of the nanovesicles. Furthermore, ethanol has a modifying effect on the structure of cornified or lipophilic domains, leading to a lowering of the lipid transition temperature. As a consequence, ethanol-based nanovesicles exhibit a softer and less rigid structure compared to liposomal nanovesicles.
One notable aspect is the influence of ethanol on the stability of nanovesicles during storage. Ethanol-based nanovesicles, characterized by a negative surface charge, possess electrostatic repelling properties that contribute to their stability. This property makes them less prone to aggregation or fusion during storage, ensuring a more stable formulation over time.20
Methods of preparation
The routine methods used for the preparation of nanovesicles were adopted among them the fowling is the popular one.
Mechanical dispersion technique
In the preparation of multilamellar vesicles, a solution of ethanolic phospholipids acts as the initial medium for dissolving drugs and terpenes or terpene mixtures. The process involves mixing and sonication for 5 min, ensuring a homogeneous dispersion. Continuous swirling with a syringe needle and the gradual addition of buffer (pH: 7.4) maintain the desired pH environment. A subsequent five-minute vortexing phase enhances homogeneity. The solution is then extruded through polycarbonate membranes of various pore sizes to form multilamellar vesicles, with repetitive piercing further refining their characteristics. This method ensures the encapsulation of drugs and terpenes within the vesicles, making them promising candidates for transdermal drug delivery systems.
Film hydration technique
The generation of invasomes using the standard film procedure involves a series of meticulously controlled steps to ensure the successful formation of these specialized vesicles. In this method, phospholipids dissolved in ethanol are combined with chloroform to create a homogenous solution. Subsequently, this mixture is subjected to a thin-layer drying process in a rotary flash evaporator, where the pressure is systematically reduced from 500 to 1 m bar at 50°C. This controlled evaporation process results in the formation of a thin lipid film.
To further refine the invasome formation, a vacuum at ambient temperature (1 m bar) is applied to the lipid film for a duration of 2 hr before nitrogen purging. Following this preparation, the film is hydrated, and the resulting solution is brought to room temperature over 30 min. This hydration step is crucial for initiating the vesicle formation process.
The sizing of the resultant vesicles is achieved using polycarbonate membranes with varied pore sizes. These membranes act as filters, allowing the vesicles to pass through while controlling their size distribution. The continuous forcing of the vesicles through polycarbonate membranes is a critical step for shaping and refining the characteristics of the invasomes.
An ultrasonic and vortex apparatus is employed throughout the process to ensure the homogeneity and stability of the invasome formulation. The ultrasonic treatment aids in dispersing any remaining aggregates and promotes the uniform distribution of vesicles, while vortexing helps maintain the desired consistency. The earlier successful trials made on nano vesicular transdermal patches are illustrated in Table 1.
Drug | Polymer | Permeability enhancers | Nano formulation |
---|---|---|---|
Felodipine | Soya Lecithin (SL) and egg lecithin. | Span 80 | Solid Lipid Nanoparticle (SLN)27 |
Nifedipine | SL, CHL, carbopol-940, and HPMC (Hydroxypropyl Methylcellulose) K-14. | Span 20, 40, 80, Tween 20, and 80. | Proniosomes28 |
Chlorpheniramine maleate | Lecithin and Polyvinyl Alcohol (PVA). | Span 80 | Proniosome29 |
Luteolin | CHL and PTC | Span 60 and labrasol | NLC30 |
Buflomedil | CHL and PTC | Span 80. | NLC31 |
Lisinopril Dihydrate | SL, HPMC-E15 and PVP. | Span 80 and Tween 80. | Transferosomes32 |
Itraconazole | Eudragit RL 100 and HPMC. | PEG-400 | Nanoparticles33 |
Losartan potassium | CHL and SL. | Span 20, Span 80, Tween 20, and Tween 80. | Nanoproniosomes34 |
Carvedilol | CHL | Tween 80 | NLC35 |
Etoricoxib | Modified Chitosan | Span 80 | NLC36 |
Tamoxifen citrate | β-cyclodextrin, eudragit-RL-100, ethyl cellulose, and HPMC K-15. | Span 80 | NLC37 |
Dapoxetine | Sodium Tripolyphosphate (TPP). | Propylene Glycol (PG), tween 80, and span 80. | Nanoparticles38 |
Ivabradine Hydrochloride | PTC | Pluronic F68 | NLC39 |
Lornoxicam | Glyceryl monostearate Triglycerides, and SL. | Tween 80 and Poloxamer 188. | NLC40 |
Ibuprofen | Cetostearyl alcohol | Tween 80 | SLN41 |
Oxaprozin | PTC and CHL | Tween 80 | SLN42 |
Aceclofenac | Cetyl alcohol and Transcutol. | Tween 80 | NLC43 |
Meloxicam | Cetyl palmitate | Tween 80 and Propylene glycol | Invasomes44 |
Meloxicam | Caprylic acid, Carrageenan, and Carbopol-940. | Tween 80 and PEG-400. | Invasomes45 |
thymol-invasions | Tween 80 | Invasomes46 | |
Isradipine | PTC | Tween 80 | Invasomes47 |
Capsaicin | Polyacrylic acid | Tween 80 and Tween 20 | NLC48 |
Simvastatin | Glyceryl dibehenate, Glyceryl behenate and Macrogol. | Poloxamer 188, Span 80, PVA, PVP and DMSO. | NLC49 |
Simvastatin | Cholesterol, Carbapol 940, Hydroxypropyl Methylcellulose (HPMC H15), sodium Carboxymethylcellulose (Na CMC). | Span60, Tween 80, PEG 400, propylene glycol. | Niosomes50 |
Fluvastatin | Span 60, Span 80 | Tween 80 | Nanovesicles51 |
lornoxicam | sodium deoxycholate and soybean phosphatidylcholine. | Limonene | Liposomes52 |
Aceclofenac | Guggul lipid, CHL. | Phosphatidylcholine (PC) and Dicetyl phosphate (DCP). | Liposomes53 |
Tropisetron hydrochloride | Egg PC | Span 60, Span 80 | Liposomes54 |
Ondansetron | Lecithin-egg PC | sodium taurocholate | Liposomes55 |
Ibuprofen | Ceramide-2 (N-stearoyl- (2S,2R,3R)-2-amino-1,3-octadecandiol nad Cholesterol | Cholesteryl-3-sulphate | Liposomes56 |
Past work done on nano vesicular transdermal patches.
Permeability enhancers used in invasomes along with mechanisms and role
Permeability enhancers are crucial components in invasomal formulations designed to enhance the transdermal delivery of drugs by facilitating their penetration through the skin’s barrier. Here, we elaborate on some commonly used permeability enhancers in invasomes:
Ethanol
Mechanism: Ethanol disrupts the lipid structure of the stratum corneum, increasing its permeability.
Role in Invasomes: Ethanol is often included in invasomal formulations to improve vesicle penetration into the skin layers, thereby enhancing drug delivery.
Terpenes
Mechanism: Terpenes, such as limonene, cineol, and eugenol, interact with stratum corneum lipids, leading to fluidization and increased permeability.
Role in Invasomes: Terpenes are essential components of invasomes, contributing to their ability to efficiently penetrate the skin and enhance drug permeation.
Phospholipids
Mechanism: Phospholipids, being natural components of cell membranes, can integrate with skin lipids, disrupting the stratum corneum structure and improving vesicle penetration.
Role in Invasomes: Phospholipids form the structural backbone of invasomes, aiding in their interaction with the skin and facilitating drug delivery.
Cholesterol
Mechanism: Cholesterol enhances the fluidity and flexibility of lipid bilayers, promoting stratum corneum permeability.
Role in Invasomes: Cholesterol is commonly included in invasomal formulations to optimize vesicle structure and improve its ability to penetrate the skin.
Sugars and Carbohydrates
Mechanism: Sugars and carbohydrates can act as penetration enhancers by interacting with stratum corneum lipids and modifying their organization.
Role in Invasomes: Invasomes may incorporate sugars or carbohydrates to enhance their interaction with skin lipids, promoting vesicle penetration.
Surfactants
Mechanism: Surfactants, like sodium lauryl sulfate, disrupt stratum corneum lipid organization, enhancing drug penetration.
Role in Invasomes: Surfactants may be included in invasomal formulations to further enhance vesicle permeation into the skin layers.
Oleic Acid
Mechanism: Oleic acid, a fatty acid, can alter the organization of stratum corneum lipids, promoting enhanced permeability.
Role in Invasomes: Oleic acid is commonly used in invasomal formulations to improve skin penetration and optimize drug delivery.
Essential Oils
Mechanism: Essential oils, containing terpenes and other bioactive compounds, can enhance skin permeation through various mechanisms.
Role in Invasomes: Essential oils with penetration-enhancing properties can be incorporated into invasomes to improve drug delivery across the skin barrier.
The combination of these permeability enhancers in invasomal formulations is carefully selected to achieve an optimal balance, addressing the challenges associated with transdermal drug delivery. This approach aims to enhance drug permeation while maintaining the stability and safety of the formulation, making invasomes a promising strategy for efficient transdermal drug delivery.
Evaluation of invasomes
The invasomes were assessed for the following tests.
Entrapment efficiency
The ultracentrifugation technique was employed as a methodological approach to assess the entrapment effectiveness of invasomes. In this experimental procedure, 1 mL of the invasomal suspension was carefully transferred to Ephendroff tubes, aiming to isolate both the unentrapped drug and the invasomes from the suspension. The tubes were then subjected to centrifugation at 15,000 revolutions per minute (rpm) under cold conditions (4°C) for 15 min, executed in two consecutive rounds.
The centrifugal force exerted during this process facilitates the separation of components based on their density. The heavier particles, such as the invasomes and any encapsulated drug, tend to sediment at the bottom of the tubes, forming a pellet. On the other hand, the lighter, unentrapped substances remain in the supernatant, the clear liquid portion above the pellet.
Following the ultracentrifugation process, spectrophotometric analysis was performed on the clear supernatant. This analytical technique involves measuring the absorbance or optical density of the solution at specific wavelengths, allowing for the quantification of the free substance present in the sample. The choice of a specific wavelength is usually determined by the known absorption characteristics of the substance under investigation.
The spectrophotometric analysis of the clear portion obtained after ultracentrifugation provides valuable insights into the entrapment effectiveness of the invasomes. By comparing the absorbance values at the specific wavelength of interest, researchers can quantify the amount of unentrapped or free substance in the supernatant. The lower the absorbance, the higher the entrapment effectiveness, as it indicates a greater proportion of the substance being encapsulated within the invasomes rather than remaining free in the solution.19,21
Surface morphology
In the experimental procedure, a small volume of the prepared sample was carefully dispensed onto a transparent glass plate. This droplet was then allowed to air dry, resulting in the formation of a thin, dry film on the glass surface. To enhance the surface conductivity of the dried sample, a layer of gold was deposited using a sputter coater. Sputter coating involves bombarding a target material (in this case, gold) with high-energy ions, causing the release of vaporized particles that subsequently condense onto the sample surface, creating a thin coating.
After the sputter coating process, the surface morphology of the prepared sample was examined under a scanning electron microscope. Scanning Electron Microscopy (SEM) is a high-resolution imaging technique that utilizes electron beams to generate detailed images of the sample surface. It provides information about the topography, structure, and morphology of the specimen at a micro- to nanoscale level.
Observing the sample under SEM allows for a detailed examination of its surface features and morphology, providing insights into the structure and characteristics of the prepared material. The gold coating enhances the sample’s conductivity, enabling a clearer and more detailed imaging under the electron microscope.
This analytical approach, combining air-drying, gold sputter coating, and SEM observation, serves as a valuable tool for characterizing the surface morphology of the prepared material. It helps researchers visualize and analyze the microstructure of the sample, providing essential information for understanding its physical properties and assessing the effectiveness of the preparation method.22
Ex vivo permeation studies
Ex vivo research conducted in compliance with the institution’s animal ethics committee involved the utilization of Franz diffusion cells to perform permeation experiments on the abdominal epidermis region of male Wistar rats. The diffusion cells, featuring a 20 mL receptor capacity and an effective surface area of 2 cm2, were employed for these experiments. Prior approval from the animal ethics committee ensured the ethical treatment of the animal subjects in the study.23,24
In the permeation experiments, the invasomal preparation was introduced into the donor compartment of the Franz diffusion cells, while the receptor medium, consisting of 20 mL pH 7.4 phosphate-buffered saline, was maintained at a physiological temperature of 37°C. The Franz diffusion cells were designed to establish a controlled environment simulating the conditions encountered during transdermal drug delivery. To maintain sink conditions and ensure the continuous availability of fresh receptor medium, aliquot quantities were periodically withdrawn and replaced with new media.25,26
The assessment of the permeation process involved measuring steady-state transdermal flow (μg/cm2/hr), permeability coefficient (cm/hr), and enhancement ratio. These crucial parameters were determined using a UV spectrometer, which enabled the quantification of the invasomal formulation’s transdermal behavior. The steady-state transdermal flow represents the rate of drug permeation through the skin over time, providing insights into the formulation’s sustained release capabilities. The permeability coefficient reflects the efficiency of the drug’s penetration through the skin, indicating the rate at which the drug crosses the skin barrier. The enhancement ratio, a comparative measure, assesses the effectiveness of the invasomal preparation in improving drug permeation compared to a control.
By employing these experimental techniques and measurements, the ex vivo research aimed to comprehensively evaluate the transdermal performance of the invasomal formulation. The results obtained from the Franz diffusion cell experiments provided valuable data on invasome-mediated drug permeation, offering insights into the formulation’s potential for effective transdermal drug delivery.57,58
Skin irritation studies
In the skin irritant experiments, three healthy rabbits were employed to assess the potential irritancy of the test formulation. The experiment involved the application of formulations on unbraided skin areas, specifically on the left dorsal surface for the test formulation and the right dorsal surface for the control formulation. To ensure a clean surface, the unbraided flesh was cleansed with a rectified spirit before the application of the formulations.
Following the application, the formulations were left in contact with the skin for a period of 24 hr. After this incubation period, the mixtures were carefully withdrawn, and the skin on both the left and right dorsal surfaces was thoroughly examined. The evaluation was conducted using the main dermal irritation index categorization, a standardized method for assessing the degree of irritation caused by a substance on the skin.
The main dermal irritation index categorization involves a systematic grading system to classify skin irritation based on specific criteria. The skin was checked for observable signs of irritation, including redness and edema. These criteria are critical indicators of skin response to the applied formulations and provide a quantitative and qualitative basis for assessing the irritant potential.
Redness, also known as erythema, is characterized by a visible reddening of the skin, while edema refers to swelling caused by an accumulation of fluid in the tissues. By evaluating these parameters, the researchers could categorize the skin irritation levels according to the predetermined index.
This experimental design allowed for a comprehensive examination of the potential irritant effects of the test formulation in comparison to the control. The careful evaluation of the skin’s response, considering both visual indicators and the main dermal irritation index categorization, provided valuable insights into the irritancy profile of the tested formulation. This information is crucial for ensuring the safety and tolerability of the formulation, especially when intended for application on the skin.59–61
CONCLUSION
Transdermal delivery, a method of administering medications through the skin for systemic distribution, offers advantages such as controlled release and avoidance of gastrointestinal degradation. Utilizing nanoformulations in transdermal drug delivery has become prominent, enhancing drug absorption and prolonging release. Nano formulations, employing nanoparticles to encapsulate drug molecules, enable improved bioavailability and therapeutic efficacy. This approach allows for the preparation of transdermal formulations with varying potencies and affinities, providing flexibility to customize drug delivery systems for specific therapeutic needs. The success of transdermal delivery depends on factors like the drug’s physicochemical properties, nanoformulation design, and skin barrier characteristics. Ongoing research explores novel nanoformulations and technologies to address challenges and enhance the efficiency of transdermal drug delivery, emphasizing the need for thorough safety and efficacy evaluations before widespread clinical application.
Cite this article
Subramaniyan G, Rubina S, Ramana BV, Stanley AM, Srinivasan D. Nano-Revolution in Transdermal Drug Delivery: A Bibliographic Compilation of Vesicular System. Int. J. Pharm. Investigation. 2024;14(2):317-26.
ABBREVIATIONS
DDS | Drug delivery system |
---|---|
TDDS | Transdermal drug delivery system |
FDA | Food and Drug Administration |
GRAS | Generally regarded as safe |
SC | Stratum corneum |
h | Hour |
min | Minutes |
rpm | Revolutions per minute |
SEM | Scanning electron microscopy |
SL | Soya lecithin |
CHL | Cholesterol |
HPMC | Hydroxy propyl methyl cellulose |
PVA | Polyvinyl alcohol |
PVP | Poly vinyl pyrrolidone |
SLN | Solid lipid nanoparticle |
TPP | Sodium tripolyphosphate |
PG | Propylene glycol |
PTC | Phosphatidylcholine |
SLN | Solid lipid nanoparticles |
DMSO | Dimethyl sulfoxide |
Na CMC | Sodium carboxy methyl cellulose |
PC | Phosphatidylcholine |
DCP | Dicetyl phosphate |
References
- Ramadon D, McCrudden MT, Courtenay AJ, Donnelly RF. Enhancement strategies for transdermal drug delivery systems: current trends and applications. Drug Deliv Transl Res. 2021:1-34. [Google Scholar]
- Jyothika LSK, Ahad HA, Haranath C, Kousar S, Sadiya SH. Types of transdermal Drug Delivery Systems: A Literature Report of the past decade. 2022 [Google Scholar]
- Swetha T, Ahad HA, Reddy KK, Sekhar A, Sivaji S, Kumar B, et al. Formulation and in vitro permeation studies of ketoprofen-Ficus reticulata fruit mucilage transdermal patches. Pharm Lett. 2010;2(6):190-9. [Google Scholar]
- Ahad HA, Ishaq BM, Shaik M, Bandagisa F. Designing and characterizing of tramadol hydrochloride transdermal patches prepared with Ficus carica fruit mucilage and povidone. Pak J Pharm Sci. 2016;29(3):945-51. [PubMed] | [Google Scholar]
- Hindustan AA, Kumar CS, Anuradha C, Reddy KK, Chandra SA. A Novel Mucilage from Ficus glomerata Fruits for Transdermal Patches: Taking Indomethacin as a Model Drug. 2011 [PubMed] | [Google Scholar]
- Sabbagh F, Kim BS. Recent advances in polymeric transdermal drug delivery systems. J Control Release. 2022;341:132-46. [PubMed] | [CrossRef] | [Google Scholar]
- Fouziya B, Abdul Ahad H, Swamy Charan D, Sri Vidya J, Chandana Reddy U, Nandini Reddy P, et al. Fabrication and evaluation of cefpodoxime proxetil niosomes. Asian J Pharm Technol. 2022;12(2):109-12. [CrossRef] | [Google Scholar]
- Sailaja K, Abdul Ahad HA, Chinthaginjala H, Gudisipalli R, Rajyalakshmi SI, Vagganagari Y, et al. Approaches to creating and past successful attempts on microspheres: A primer for aspiring researchers. Res J Pharm Dosage Forms Technol. 2022;14(3):245-8. [CrossRef] | [Google Scholar]
- Yilmaz EG, Ece E, Erdem Ö, Eş I, Inci F. A Sustainable Solution to Skin Diseases: Ecofriendly Transdermal Patches. Pharmaceutics. 2023;15(2):579 [PubMed] | [CrossRef] | [Google Scholar]
- Balavigneswaran CK, Jaiswal V, Venkatesan R, Karuppiah PS, Sundaram MK, Vasudha TK, et al. Mussel-inspired adhesive hydrogels based on laponite-confined dopamine polymerization as a transdermal patch. Biomacromolecules. 2023;24(2):724-38. [PubMed] | [CrossRef] | [Google Scholar]
- Gadag S, Narayan R, Nayak Y, Garg S, Nayak UY. Design, development and evaluation of resveratrol transdermal patches for breast cancer therapy. Int J Pharm. 2023;632:122558 [PubMed] | [CrossRef] | [Google Scholar]
- Matos CM. Liposomes: the brave Old World. Int J Mol Sci. 2023;24(5):4343 [PubMed] | [CrossRef] | [Google Scholar]
- Badran MM, Alouny NN, Aldosari BN, Alhusaini AM, Abou El Ela AES. Transdermal glipizide delivery system based on chitosan-coated deformable liposomes: development, ex vivo, and in vivo studies. Pharmaceutics. 2022;14(4):826 [PubMed] | [CrossRef] | [Google Scholar]
- Kuznetsova DA, Vasilieva EA, Kuznetsov DM, Lenina OA, Filippov SK, Petrov KA, et al. Enhancement of the transdermal delivery of nonsteroidal anti-inflammatory drugs using liposomes containing cationic surfactants. ACS Omega. 2022;7(29):25741-50. [PubMed] | [CrossRef] | [Google Scholar]
- Shang H, Younas A, Zhang N. Recent advances on transdermal delivery systems for the treatment of arthritic injuries: from classical treatment to nanomedicines. Wiley Interdiscip Rev Nanomed Nanobiotechnology. 2022;14(3):e1778 [PubMed] | [CrossRef] | [Google Scholar]
- Huang C, Gou K, Yue X, Zhao S, Zeng R, Qu Y, et al. A novel hyaluronic acid-based dissolving microneedle patch loaded with ginsenoside Rg3 liposome for effectively alleviate psoriasis. Mater Des. 2022;224:111363 [CrossRef] | [Google Scholar]
- Ni Y, Zhao W, Cheng W, Deng C, Ying Z, Li L, et al. Lipopeptide liposomes-loaded hydrogel for multistage transdermal chemotherapy of melanoma. J Control Release. 2022;351:245-54. [PubMed] | [CrossRef] | [Google Scholar]
- El-Tokhy FSe, Abdel-Mottaleb MM, El-Ghany EA, Geneidi AS. Design of long acting invasomal nanovesicles for improved transdermal permeation and bioavailability of asenapine maleate for the chronic treatment of schizophrenia. International Journal of Pharmaceutics. 2021;608:121080 [PubMed] | [CrossRef] | [Google Scholar]
- Kumar B, Manchanda S. Development of eucalyptol enriched nanovesicles for better transdermal delivery of curcumin: preparation, characterisation and ex vivo skin analysis. Nanomed J. 2022;9(3) [PubMed] | [CrossRef] | [Google Scholar]
- de Matos SP, Teixeira HF, de Lima ÁAN, Veiga-Junior VF, Koester LS. Essential oils and isolated terpenes in nanosystems designed for topical administration: a review. Biomolecules. 2019;9(4):138 [PubMed] | [CrossRef] | [Google Scholar]
- Chacko IA, Ghate VM, Dsouza L, Lewis SA. Lipid vesicles: A versatile drug delivery platform for dermal and transdermal applications. Colloids Surf B Biointerfaces. 2020;195:111262 [PubMed] | [CrossRef] | [Google Scholar]
- Chede LS, Wagner BA, Buettner GR, Donovan MD. Electron spin resonance evaluation of buccal membrane fluidity alterations by sodium caprylate and L-menthol. Int J Mol Sci. 2021;22(19):10708 [PubMed] | [CrossRef] | [Google Scholar]
- Pathak K, Vaidya A, Sharma V. Confronting penetration threshold via fluidic terpenoid nanovesicles. Curr Drug Deliv. 2018;15(6):765-76. [PubMed] | [CrossRef] | [Google Scholar]
- El-Nabarawi MA, Shamma RN, Farouk F, Nasralla SM. Dapsone-loaded invasomes as a potential treatment of acne: preparation, characterization, and in vivo skin deposition assay. AAPS PharmSciTech. 2018;19(5):2174-84. [PubMed] | [CrossRef] | [Google Scholar]
- Kumar B, Sahoo PK, Manchanda S. Formulation, characterization and ex vivo study of curcumin Nano-invasomal gel for enhanced transdermal delivery. OpenNano. 2022;7:100058 [CrossRef] | [Google Scholar]
- Mancuso A, Cristiano MC, Fresta M, Paolino D. The challenge of nanovesicles for selective topical delivery for acne treatment: enhancing absorption whilst avoiding toxicity. Int J Nanomedicine. 2020;15:9197-210. [PubMed] | [CrossRef] | [Google Scholar]
- Teaima MH, Eltabeeb MA, El-Nabarawi MA, Abdellatif MM. Utilization of propranolol hydrochloride mucoadhesive invasomes as a locally acting contraceptive: in vitro, ex vivo, and in vivo evaluation. Drug Deliv. 2022;29(1):2549-60. [PubMed] | [CrossRef] | [Google Scholar]
- Kumar B, Pandey M, Aggarwal R, Sahoo PK. A comprehensive review on invasomal carriers incorporating natural terpenes for augmented transdermal delivery. Future J Pharm Sci. 2022;8(1):50 [CrossRef] | [Google Scholar]
- Shailaja AK, Afreen U. Formulation and evaluation of naproxen sodium loaded invasomes for topical delivery. Curr Nanomed (Formerly: Recent Patents on Nanomedicine). 2022;12(1):32-43. [CrossRef] | [Google Scholar]
- Verma H, Pal DP, Joshi D. Formulation, development and evaluation of invasomes loaded gel for fungal treatment. Sch Acad J Pharm. 2022;11(7):105-8. [CrossRef] | [Google Scholar]
- Ananda PWR, Elim D, Zaman HS, Muslimin W, Tunggeng MGR, Permana AD, et al. Combination of transdermal patches and solid microneedles for improved transdermal delivery of primaquine. Int J Pharm. 2021;609:121204 [PubMed] | [CrossRef] | [Google Scholar]
- Yusuf M, Sharma V, Pathak K. Nanovesicles for transdermal delivery of felodipine: development, characterization, and pharmacokinetics. Int J Pharm Investig. 2014;4(3):119-30. [PubMed] | [CrossRef] | [Google Scholar]
- Yasam VR, Jakki SL, Natarajan J, Venkatachalam S, Kuppusamy G, Sood S, et al. A novel vesicular transdermal delivery of nifedipine-preparation, characterization and in vitro/in vivo evaluation. Drug Deliv. 2016;23(2):619-30. [PubMed] | [CrossRef] | [Google Scholar]
- Varshosaz J, Pardakhty A, Mohsen SM, Baharanchi H. Sorbitan monopalmitate-based proniosomes for transdermal delivery of chlorpheniramine maleate. Drug Deliv. 2005;12(2):75-82. [PubMed] | [CrossRef] | [Google Scholar]
- Imam SS, Alshehri S, Altamimi MA, Hussain A, Alyahya KH, Mahdi WA, et al. Formulation and evaluation of luteolin-loaded nanovesicles: in vitro physicochemical characterization and viability assessment. ACS Omega. 2022;7(1):1048-56. [PubMed] | [CrossRef] | [Google Scholar]
- Akhtar N. Vesicles: a recently developed novel carrier for enhanced topical drug delivery. Curr Drug Deliv. 2014;11(1):87-97. [PubMed] | [CrossRef] | [Google Scholar]
- Alfatama M, Rashid ZM. Drug delivery using nanomaterials. ;2022:49-84. [PubMed] | [CrossRef] | [Google Scholar]
- Rao MT, Rao YS, Ratna J V, Kumari PvK. Characterization and ex vivo studies of nanoparticle incorporated transdermal patch of itraconazole. Indian J Pharm Sci. 2020;82(5):809-18. [CrossRef] | [Google Scholar]
- Sabareesh M, Yanadaiah JP, Sekhar KBC. Formulation development, ex vivo evaluation and in vivo antihypertensive study of losartan potassium loaded nanoproniosomal gel: A novel vesicular approach for transdermal delivery. Res J Pharm Technol. 2021;14(3):1423-30. [CrossRef] | [Google Scholar]
- Maan S, Khar RK, Mazumder R, Yadav N, Khan UA. Fabrication and evaluation of controlled release transdermal drug delivery system of carvedilol using design Expert® software for the management of hypertension. J Young Pharm. 2022;14(3):295-301. [CrossRef] | [Google Scholar]
- Wahid A, Sridhar BK, Shivakumar S. Preparation and evaluation of transdermal drug delivery system of etoricoxib using modified chitosan. Indian J Pharm Sci. 2008;70(4):455-60. [PubMed] | [CrossRef] | [Google Scholar]
- Adhyapak A, Desai B. Preparation and in vitro characterization of the transdermal drug delivery system containing tamoxifen citrate for breast cancer. Asian J Pharm. 2011;5(1) [CrossRef] | [Google Scholar]
- Ahmed TA, Alay AMS, Okbazghi SZ, Alhakamy NA. Two-step optimization to develop a transdermal film loaded with dapoxetine nanoparticles: a promising technique to improve drug skin permeation. Dose-Response. 2020;18(2):1559325820923859 [PubMed] | [CrossRef] | [Google Scholar]
- Naguib MJ, Elsayed I, Teaima MH. Simultaneous optimization of oral and transdermal nanovesicles for bioavailability enhancement of ivabradine hydrochloride. Int J Nanomedicine. 2021;16:2917-31. [PubMed] | [CrossRef] | [Google Scholar]
- Gao S, Tian B, Han J, Zhang J, Shi Y, Lv Q, et al. Enhanced transdermal delivery of lornoxicam by nanostructured lipid carrier gels modified with polyarginine peptide for treatment of carrageenan-induced rat paw edema. Int J Nanomedicine. 2019;14:6135-50. [PubMed] | [CrossRef] | [Google Scholar]
- Elkomy MH, Elmenshawe SF, Eid HM, Ali AM. Topical ketoprofen nanogel: artificial neural network optimization, clustered bootstrap validation, and in vivo activity evaluation based on longitudinal dose response modeling. Drug Deliv. 2016;23(9):3294-306. [PubMed] | [CrossRef] | [Google Scholar]
- Pham CV, Van MC, Thi HP, Thanh C, Ngoc BT, Van BN, et al. Development of ibuprofen-loaded solid lipid nanoparticle-based hydrogels for enhanced in vitro dermal permeation and in vivo topical anti-inflammatory activity. J Drug Deliv Sci Technol. 2020;57:101758 [CrossRef] | [Google Scholar]
- Mennini N, Cirri M, Maestrelli F, Mura P. Comparison of liposomal and NLC (nanostructured lipid carrier) formulations for improving the transdermal delivery of oxaprozin: effect of cyclodextrin complexation. Int J Pharm. 2016;515(1-2):684-91. [PubMed] | [CrossRef] | [Google Scholar]
- Sinha P, Srivastava N, Rai VK, Mishra R, Ajayakumar PV, Yadav NP, et al. A novel approach for dermal controlled release of salicylic acid for improved anti-inflammatory action: combination of hydrophilic-lipophilic balance and response surface methodology. J Drug Deliv Sci Technol. 2019;52:870-84. [CrossRef] | [Google Scholar]
- Khurana S, Bedi PM, Jain NK. Preparation and evaluation of solid lipid nanoparticles based nanogel for dermal delivery of meloxicam. Chem Phys Lipids. 2013;175-176:65-72. [PubMed] | [CrossRef] | [Google Scholar]
- Kaltschmidt BP, Ennen I, Greiner JFW, Dietsch R, Patel A, Kaltschmidt B, et al. Preparation of terpenoid-invasomes with selective activity against S. aureus and characterization by cryo transmission electron microscopy. Biomedicines. 2020;8(5):105 [PubMed] | [CrossRef] | [Google Scholar]
- Qadri GR, Ahad A, Aqil M, Imam SS, Ali A. Invasomes of isradipine for enhanced transdermal delivery against hypertension: formulation, characterization, and in vivo pharmacodynamic study. Artif Cells Nanomed Biotechnol. 2017;45(1):139-45. [PubMed] | [CrossRef] | [Google Scholar]
- Arunprasert K, Pornpitchanarong C, Piemvuthi C, Siraprapapornsakul S, Sripeangchan S, Lertsrimongkol O, et al. Nanostructured lipid carrier-embedded polyacrylic acid transdermal patches for improved transdermal delivery of capsaicin. Eur J Pharm Sci. 2022;173:106169 [PubMed] | [CrossRef] | [Google Scholar]
- Brito Raj S, Chandrasekhar KB, Reddy KB. Formulation, in vitro and in vivo pharmacokinetic evaluation of simvastatin nanostructured lipid carrier loaded transdermal drug delivery system. Future J Pharm Sci. 2019;5(1):1-14. [PubMed] | [CrossRef] | [Google Scholar]
- Salem HF, Kharshoum RM, Abou-Taleb HA, Farouk HO, Zaki RM. Fabrication and appraisal of simvastatin via tailored niosomal nanovesicles for transdermal delivery enhancement: in vitro and in vivo assessment. Pharmaceutics. 2021;13(2):138 [PubMed] | [CrossRef] | [Google Scholar]
- El Menshawe SF, Nafady MM, Aboud HM, Kharshoum RM, Elkelawy AMMH, Hamad DS, et al. Transdermal delivery of fluvastatin sodium via tailored spanlastic nanovesicles: mitigated Freund’s adjuvant-induced rheumatoid arthritis in rats through suppressing p38 MAPK signaling pathway. Drug Deliv. 2019;26(1):1140-54. [PubMed] | [CrossRef] | [Google Scholar]
- Ahmed S, Kassem MA, Sayed S. Bilosomes as promising nanovesicular carriers for improved transdermal delivery: construction, in vitro optimization, ex vivo permeation and in vivo evaluation. Int J Nanomedicine. 2020;15:9783-98. [PubMed] | [CrossRef] | [Google Scholar]
- Gaur PK, Mishra S, Aeri V. Formulation and evaluation of guggul lipid nanovesicles for transdermal delivery of aceclofenac. Scientific World Journal. 2014;2014:534210 [PubMed] | [CrossRef] | [Google Scholar]
- Abdel-Messih HA, Ishak RAH, Geneidi AS, Mansour S. Tailoring novel soft nanovesicles ‘Flexosomes’ for enhanced transdermal drug delivery: optimization, characterization and comprehensive exvivo-in vivo evaluation. Int J Pharm. 2019;560:101-15. [PubMed] | [CrossRef] | [Google Scholar]
- Habib BA, Sayed S, Elsayed GM. Enhanced transdermal delivery of ondansetron using nanovesicular systems: fabrication, characterization, optimization and ex vivo permeation study-Box-Cox transformation practical example. Eur J Pharm Sci. 2018;115:352-61. [PubMed] | [CrossRef] | [Google Scholar]
- Gaur PK, Mishra S, Purohit S, Kumar Y, Bhandari A. Development of a new nanovesicle formulation as transdermal carrier: formulation, physicochemical characterization, permeation studies and anti-inflammatory activity. Artif Cells Nanomed Biotechnol. 2014;42(5):323-30. [PubMed] | [CrossRef] | [Google Scholar]