ABSTRACT
Background
Cancer is the most lethal disease in humans, accounting for one out of every six fatalities. The most frequent type of cancer among women is breast cancer, followed by cervix cancer. Iron oxide nanoparticles, due to their superparamagnetic traits, are one of the most widely used nanomaterials for diagnosing and treating breast cancer. For site-specific medication delivery, biotin was coupled with magnetite nanoparticles.
Materials and Methods
The dextran-coated IONP was synthesized by a simple coprecipitation process and biotin was attached to the surface of dextran via a carboxylic/amine group. X-ray Diffractometer, Thermal gravimetric Analysis, Fourier Transformed Infrared Spectrometer, Atomic Force Microscopy and Vibrating Sample Magnetometer were used to investigate the structural, morphological, and magnetic properties of the produced materials.
Results and Discussion
The iron oxide nanoparticle particle size was determined to be 325 nm. The MTT assay of IONPs and dextran-coated biotin-conjugated IONPs with low IC50 values of 24.18 μg/mL and 1.66 μg/mL exhibit cytotoxicity toxicity against MCF 7 cells, whereas the cytotoxicity of biotin-linked IONPs higher when compared with pure iron oxide.
Conclusion
Iron oxide nanoparticle and dextran-coated biotin-conjugated iron oxide nanoparticle results confirmed the anti-breast cancer efficacy on human breast cancer cells by causing effective cytotoxicity and ensuring the drug delivery to a specific site.
INTRODUCTION
Cancer is the most disastrous disease in humans. The primary warning sign of breast carcinoma is a bulge that seems distinct from other parts of the breast tissue. Carcinoma of the breast can also be acknowledged by tumors in the armpit lymph nodes. In the fight against cancer, Hyperthermia, radiation, gene therapy, chemotherapy, and other treatments have all been employed.1
Theranostic nanomedicine has the potential to systemically deliver infection-specific diagnosis and treatment. Utilizing the appropriate carrier molecule could prevent interactions with normal cells and cause only the tumor cells to be eliminated.1 To improve survival, early identification, diagnosis, and treatment are crucial. Modern approaches for diagnosing and treating breast cancer are now available with unique advancements made possible by nanotechnology.
Iron oxides are employed across multiple technical applications, including magnetic recording media, magnetic inks, ferrofluids, catalysts, and biomedical applications (for example, magnetic resonance imaging, immunoassay, site-specific drug delivery for diseases such as carcinoma, hyperthermia, gene therapy, DNA separation, and cell sorting).2 The three prevalent kinds of iron are magnetite (Fe3O4), maghemite (-Fe2O3) and hematite (-Fe2O3).3
Among the oxides utilized in biomedical applications, iron oxide has the highest intrinsic magnetic response; it has also been given FDA approval for usage in people. Iron oxide nanoparticles and immune cells can combine to cause an anti-tumor immune response.4
In cancer detection and therapy, inorganic nanoparticles are used. These nanomaterials can also be linked with tumor-specific ligands and used to deliver highly selective chemotherapeutic or hormonal medicines. These inorganic particles may cling to cancer cells and become magnetized after being exposed to a magnetic field. With the help of an external magnet, IONP can be amalgamated with chemotherapy agents, immunoglobulins nucleic acids, enzymes, and proteins before being transported to the desired site.5
Particles in various biomedical applications must be capped with a biodegradable substance and a unique kind of surface-coating chemistry to bind biological molecules such as medicines, enzymes, proteins, and antibodies. Surface functionalization not only expands surface conjugation options but also improves biocompatibility by reducing particle aggregation and surface oxidation.6
Dextran is an organic substance that is commonly utilized in medical fields to coat nanoparticles to prevent magnetic nanoparticle aggregation and toxicity. Some vital vitamins, such as folic acid, vitamin B12, biotin, and riboflavin, are purposefully chosen to target cancer cells.7 Biotin-targeted systems are unquestionably high carcinogenic than folate or vitamin B12-targeted systems. The Sodium-Dependent Multivitamin Transporter (SMVT) has been identified as the sole biotin vehicle in Ovarian (OV 2008, ID8), Leukemic (L1210FR), mastocytoma (P815), Colon (Colo-26), Lung (M109), Renal (RENCA, RD0995) and Breast (4T1, JC, MMT06056) cancer cell lines.8
In this investigation, dextran-coated biotin-conjugated IONPs were produced and screened for in vitro breast cancer activity.
DOE for Iron Oxide Nanoparticles Optimization
A Design of Experiments (DOE) is utilized to reduce the massive number of tests demanded since there are a multitude of synthesis variables or factors. 22 factorial designs were put to use for statistical formulation optimization. pH and temperature are the independent variables at two different levels that were investigated. The dependent variables are the size of the particles (Y1) and zeta potential (Y2). The performing values were subjected to a Design expert software and anatomized (Table 1).9
Runs | pH | Temperature (°C) | Particle size (nm) | Zeta potential (mv) |
---|---|---|---|---|
1 | 6.5 | 60 | 403.25±0.66 | -21.26±1.01 |
2 | 11 | 25 | 523.04±0.93 | -24.6±0.36 |
3 | 11 | 60 | 255.64±0.6 | -23.8±0.173 |
4 | 6.5 | 25 | 677.31±1.10 | -22.59±0.16 |
MATERIALS AND METHODS
Materials
Ferric chloride anhydrous (Spectrum Reagents and Chemicals, Kerala), Ferrous Sulphate (Hi Media Laboratories, Bangalore), Potassium Hydroxide pellets (Thomas Baker Private Limited, Bangalore), Urea, Dextran (Hi Media Laboratories, Bangalore), Dimethyl sulfoxide (Sigma Aldrich, USA) and Deionized water as starting precursors. Biotin (Sigma Aldrich),1-ethyl- 3(3-dimethylaminopropyl) Carbodiimide hydrochloride (Sigma Aldrich), N-hydroxy succinimide (Sigma Aldrich).
Methods
Synthesis of Dextran coated iron oxide nanoparticle
Simple coprecipitation techniques were used for fabricating the IONPS. 100 mL of distilled water, 2.7 g of Ferric chloride anhydrous in nature, and 1 g of ferrous sulfate were submerged to generate a light-yellow solution. In 100 mL of distilled water, 2.8 g potassium hydroxide and 0.5 g urea were dissolved and added to the above solution until it reached pH 11, then agitated for 30 min at 60°C. The solution was then gradually supplemented with 2.5 g of dextran dispersed in 100 mL of distilled water. To minimize phase transition and to increase the stability of magnetite nanoparticles, nitrogen gas was continually inflated in the solution to eliminate unattached oxygen. This solution was held at 80-90°C with perpetual stirring for 90 min before naturally cooling to room temperature. Using an efficient external magnet, the resultant black precipitate IONP was recovered. To remove adhering dextran, the supernatant was rinsed with distilled water multiple times. This was allowed to dry at ambient temperature for several hours before being further characterized.10
Biotin conjugation
Using the nanoprecipitation process, biotin-attached nanoparticles were produced. Biotin is water-insoluble but alkali (5.5 M potassium hydroxide) soluble. In 3 mL of 5.5 M potassium hydroxide, 10 mg of biotin was dissolved. The dextran-coated IONP was then dissolved in 30 mL of distilled water and DMSO. The magnetite nanoparticles were treated with 10 mg of N-Hydroxy Succinimide (NHS) and 1-Ethyl-3-(3-Dimethylaminopropyl) Carbodiimide (EDC) for 30 min. The previous combination was then mixed with the biotin solution and the two were combined for 24 hr at ambient temperature. By adding potassium hydroxide solution, the pH of the resultant mixture was raised to 10. As an outcome, the color has become black. To remove any disconnected biotin, the black residue was washed with distilled water. The drug-loaded magnetite nanoparticles were then gathered outside with a magnet and dried with a freeze-dryer. These drug-conjugated magnetite nanoparticles were stored at temperatures below room temperature for future research.10
Characterization
Particle size
Particle size of IONP and biotin-conjugated IONP were analyzed by Malvern Zeta sizer.10
Surface charge
The surface charge of ferromagnetic nanoparticles was assessed through the Malvern Zeta sizer.10
Fourier transform infrared spectroscopy
A viable approach to assess functional group chemical adsorption on magnetite nanoparticles is FT-IR. The medication, excipients, and formulation physical mixes go through FT-IR testing using the KBr press pellet technique.11
Morphology
Atomic Force Microscopy (AFM) was used to assess the morphology of IONPs and biotin-linked IONPs. The surface morphology and roughness of nanomaterials were investigated using AFM. A modest quantity of the nanoparticle formulation (5L) was introduced over a glass plate to form the sample, which was then used as the basis for subsequent research.12
Magnetic properties
A vibrating sample magnetometer can be employed to measure both the intensity and the direction of a magnetic field. The magnetic properties of electromagnetically delicate (low coercivity) and firm (high coercivity) materials, like solids as well, pulverizers, isolated crystals, thin films, and liquids, are checked using VSM. The magnetic properties of IONP, dextran-coated IONP, and biotin-conjugated IONP were examined using the hysteresis loop at ambient temperature.13
X-ray Diffraction
An X-ray diffractometer is used for assessing the crystallography of a substance. On a PANalytical X’PERT PRO powder, XRD measurements were made using monochromatic Cu Kα1 (λ=1.54056 Å) radiation.14
Thermal gravimetric analysis
TGA analyses changes in a material’s physical and chemical attributes as a function of temperature that falls between 25 towards 150°C. The second-order phase transitions, such as vaporization, sublimation, absorption and desorption (or chemisorption), desolvation, and decomposition, are physical and chemical phenomena that are determined by TGA. TGA is frequently used to assess certain properties of materials that show mass loss or gain as a result of oxidation, breakdown, or moisture loss.15
In vitro cytotoxicity activity in MCF-7 cells
The lethality of iron oxide and biotin-connected IONP has been assessed using the MTT (3-(4,5-dimethylthiahiazol-2-yl)- 2,5-diphenyl tetrazolium) assay. First, 1×104 MCF-7 cells were propagated in 96-well plates for 48 hr. Following that, the MCF-7 cells were exposed for 24 hr at 37°C to control pure iron oxide and biotin-conjugated Fe3O4 nanoparticles. The control group consisted of pretreated MCF-7 cells. Following incubation, each well acquired 100 μL of MTT reagent (5 mg/mL). After that, the plate was kept warm for 4 hr at 37°C and 5% CO2. Following their formation, the formazan crystals were disseminated in a 100 μL DMSO solution. The absorbance ratio was used to calculate the optical density and percentage of living cells.16
Stability studies
Particle size, PDI, and surface charge have been examined to assess formulation stability. The optimized formulation was kept in a stability chamber (8±2°C), room temperature (25±3°C), and refrigerated temperature (40±2°C). At 1 and 2 months, the formulation’s particle size and Zeta potential were assessed.
RESULTS AND DISCUSSION
DOE for iron oxide nanoparticle optimization
To determine the optimal criterion for producing IONPs, 22 factorial designs with four formulations were created using Design Expert software. Design expert software was used to apply desirable constraints on particle size and zeta potential.
From Table 1, the mixture with pH 11 and a temperature 60°C has a particle size of about 256 nm and a surface charge of about -23.4 mV, and the mixture with pH 6.5 and a temperature 60°C has a particle size of about 404 nm and a surface charge of about -21.8 mV. Hence pH influences the zeta potential and particle size of the formulation. An increase in pH has desirable particle size.
The second-order regression equation was adopted in a numerical optimization study for the desired function to produce an optimum formulation with the required response. The chosen parameters and each response are linked using a second-order polynomial equation produced in terms of coded components.
The polynomial equation for particle size is,
Positive concentrations of ‘A’ (pH) and ‘B’ (temperature) have an additive effect on particle dimension, whereas low concentrations have a diabolical impact on response. An upsurge in pH, according to this equation, has beneficial effects on the size of particles (desirable particle size).
The polynomial equation for zeta potential is,
According to this equation. Increases in pH have a detrimental impact on zeta potential (Stable zeta potential).
To offer an ideal formulation with the appropriate response based on the appealing criteria for particle dimension, surface charge, and particle shape, the design expert programme did a numerical optimization analysis using the desirability factor. The overall desirability of the formulation will be 0.9 with a pH 8.75 and a temperature of 60°C.
The enhanced formulation originated from chemical reduction, which has a particle size of 326±1 nm and a surface charge of -23.45±1.2 mV.
Particle size
Dynamic Laser Scattering (DLS) was used to explore the size of dextran-coated Fe3O4 IONPs and biotin-linked Fe3O4 nanoparticles. The dextran-coated IONPs possessed a size of 325 nm, whereas the biotin-linked Fe3O4 nanomaterials displayed a size of 340 nm. The presence of a conjugated ligand explains the variation. The raised average size is most likely the result of efficient biotin conjugation on dextran-coated IONP via the EDC/NHS reaction.
Zeta potential
Because of the deprotonated surface, the dextran-coated IONPs have a negative surface charge of -25.6 mV. The significant electrostatic collision between the biotin and the surface groups of the dextran via carboxyl molecules offers the biotin-connected iron oxide nanoparticles a positive surface charge of 20.14 mV. The negatively charged surface of the cancer cell immediately interacts with this positively charged surface, which may aid in medicine delivery efficiency. It may also help to promote drug-deep penetration into cancer cells to achieve homogenous allocation. The change in surface charge also demonstrates that biotin molecules were properly conjugated on the surface of the magnetite nanoparticles.
Fourier Transform Infrared Spectroscopy
FT-IR is an excellent approach for identifying functional group chemical adsorption on magnetite nanoparticles. FT-IR spectra of biotin-conjugated iron oxide nanoparticle. The broad absorption peak at 1269.20cm-1 represents the C-N stretching and shows the presence of an amine group. The broad peaks at 891cm-1 represent the C-C l stretching showing the presence of halo compound. The broad peaks at 2915.50 cm-1 represent the 0-H stretching showing the presence of alcohol. The broad peaks at 798.56cm-1 represent the C=C bending in the presence of an alkane compound. The IR spectrum of the physical mixture confirming Biotin conjugated dextran-coated IONP not have any incompatibility with the excipients.
Atomic Force Microscopy
The average height of IONP and biotin-conjugated nanoparticles was found to be 43.51 nm and 46.18 nm. IONP nanoparticles and biotin conjugated had an average diameter 87.14 nm and 90.96 nm. The average height and average diameter of IONP nanoparticles and biotin-conjugated iron oxide nanoparticles differ much and hence not spherical.
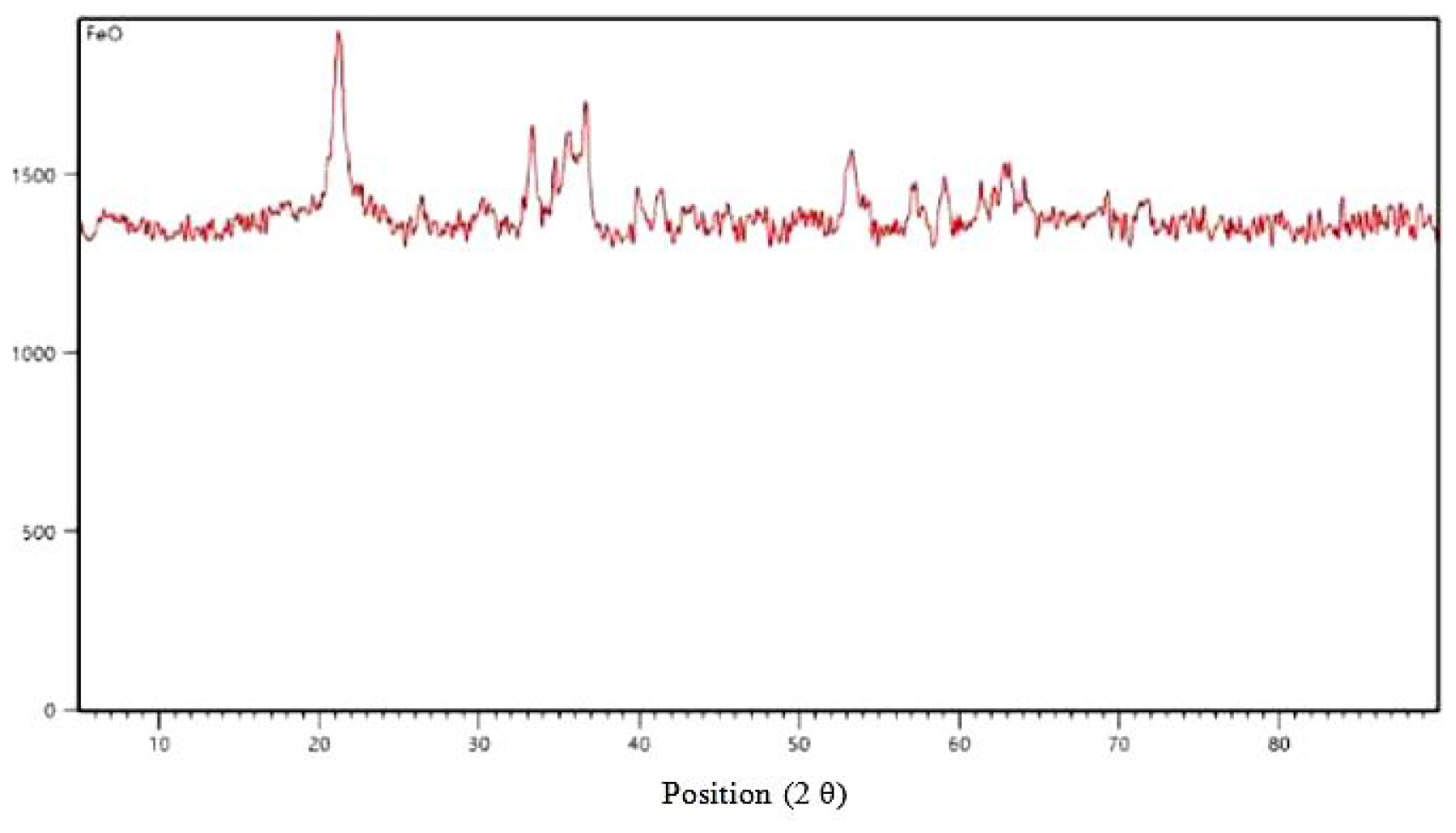
Figure 1:
XRD of Iron oxide nanoparticle.
Magnetic properties
The pure iron oxide nanoparticles showed Ms value of 19 emu/g which is lower than the standard value of 92 emu/g. The spin canting effects and the magnetic moment are easily reversed when the nanoparticle from bulk particles. After being coated with dextran, the Ms value is further decreased to 13.5 emu/g. This was due to the presence or coverage of non-magnetic materials on the surface of magnetic nanoparticles. Both pure and dextran-coated sample shows negligible coercivity and remanence with superparamagnetic behavior. In contrast, the biotin conjugation with dextran-coated magnetic nanoparticles leads to different trends of ferromagnetic behavior (Ms of 16 emu/g) with higher remanence and lower coercivity instead of superparamagnetic behavior.
X-ray diffraction
XRD measurement revealed the crystalline/amorphous character of Fe3O4 nanoparticles. The diffraction peaks were all orthorhombic magnetite nanoparticle structures (Figure 1) . The XRD pattern’s lack of any additional peaks serves as evidence of the magnetite nanoparticle’s purity. The peaks’ prominent intensity and enlargement confirm the crystalline character of the produced magnetite nanoparticles.
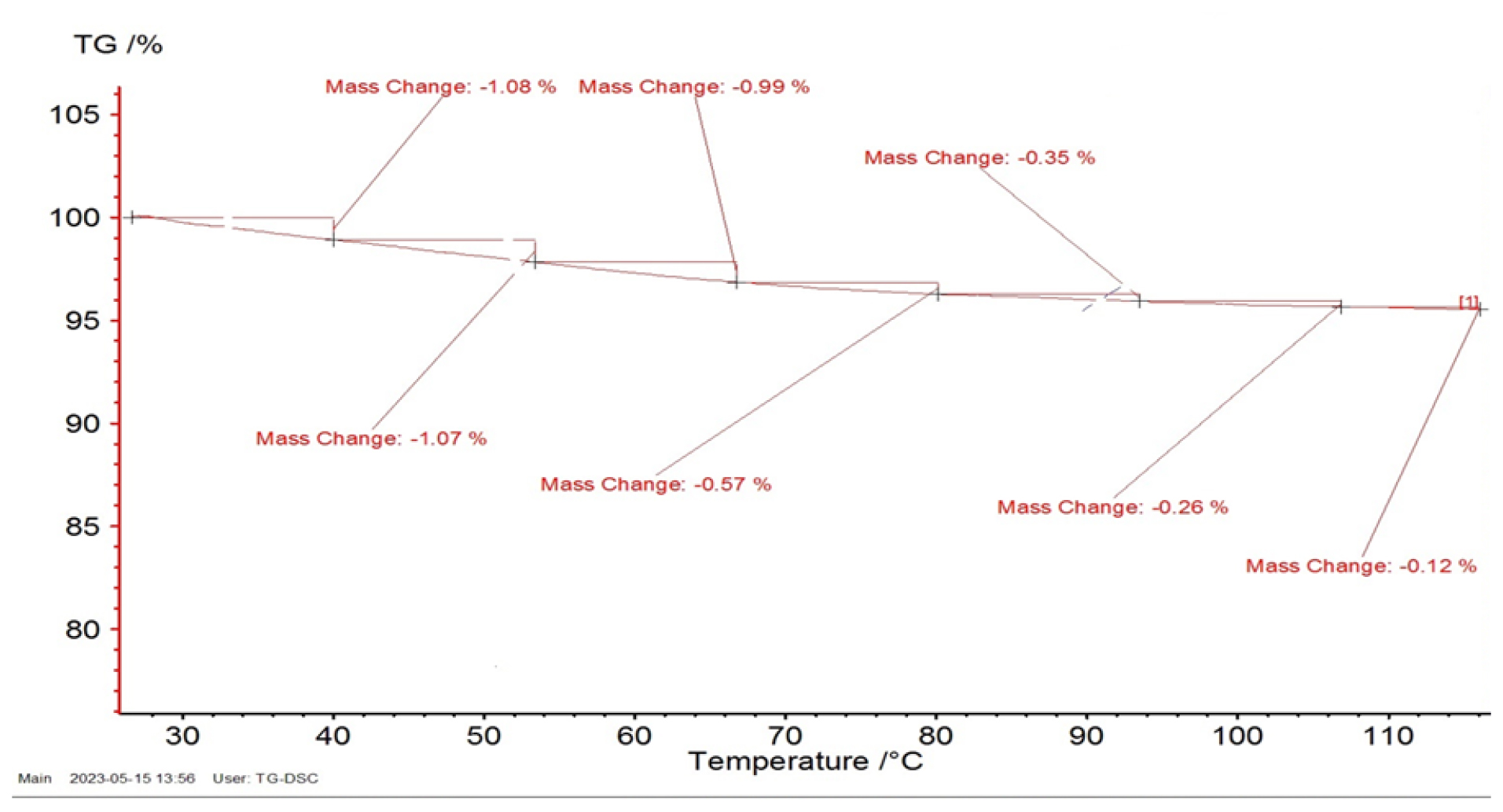
Figure 2:
TGA of Biotin conjugated dextran coated Iron oxide nanoparticle.
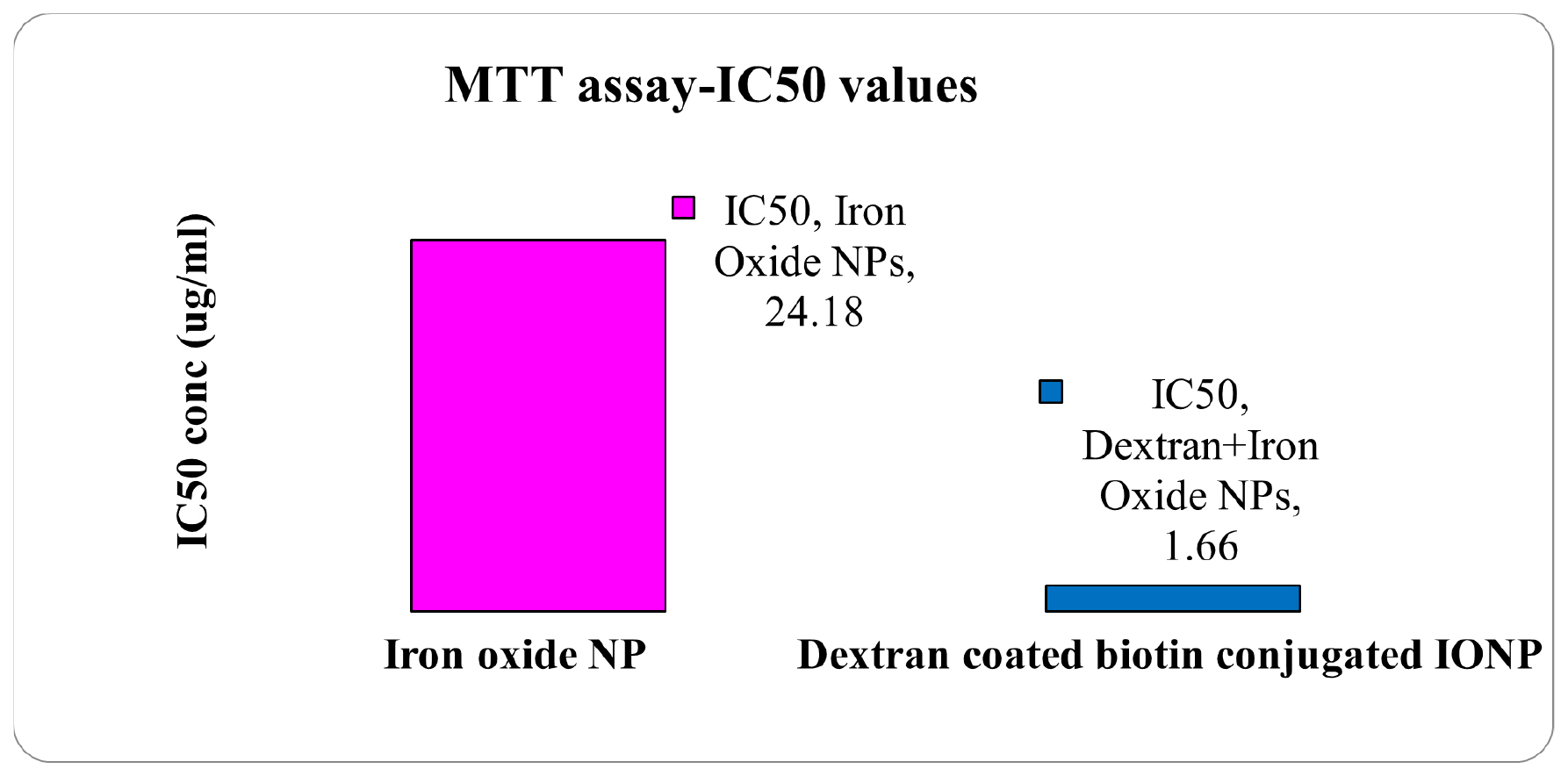
Figure 3:
Comparative IC50 values of IONP and Biotin conjugated dextran Coated IONP against MCF7 cells.
Thermal gravimetric analysis
The thermogravimetric analysis was performed between 25 and 150°C. Weight loss occurs at different times as the temperature rises. IONP loses 4% of its weight, while dextran-coated IONP loses 3%. The first weight loss occurs between 30°C and 44°C and it is approximately 1.56% due to the removal of water from the surface of Biotin-linked dextran-coated IONPs (Figure 2). Another weight loss of 5.04% was noticed as the temperature reached 110°C due to the elimination of organic chemicals present in the nanoparticles. After 110°C, no additional changes were observed, indicating that the biotin-conjugated dextran-coated IONPs were exceptionally thermally stable.
MTT cytotoxicity
The MTT assay findings indicate that the specified nanoformulations, IONPs, and Dextran Coated biotin-conjugated IONPs were effectively cytotoxic in nature on human breast carcinoma with low IC50 values of 24.18 μg/mL (24) and 1.66 μg/ mL (2) after the incubation period of 24 hr respectively (Figure 3). Overall, the observed results confirmed the anti-breast cancer efficacy of both nanoformulations on human breast cancer cells by causing effective toxicity. Among both, Dextran coated Biotin conjugated Iron Oxide NPs have a lower IC50 value than the Iron Oxide NPs. And also confirmed that Dextran coated biotin conjugated Iron Oxide NPs have less toxicity potential than the Iron Oxide NPs.
Stability studies
At 1 and 2 months, the formulations’ zeta potential and particle size were evaluated and compared in a room, refrigerated temperature, and a stability chamber. When compared to the formulation held in the stability chamber, the difference in particle size and surface charge between room and refrigerated formulations is relatively small (Table 2). The formulation is more stable when stored at ambient and refrigerated temperatures than when stored in a chamber. High humidity could be the cause of decreased formulation stability.
Months | Temperature condition | Particle Size (nm) | Zeta Potential (mV) |
---|---|---|---|
0 Month | Room temperature (25°C) | 341±0.7 | 21.4±1.2 |
Refrigerated temperature (40°C) | 341.6±2.08 | 22±1.8 | |
Stability chamber (8°C) | 340.6±1.15 | 22.4±0.4 | |
1 Month | Room temperature (25°C) | 342.3±0.5 | 22.6±0.4 |
Refrigerated temperature (40°C) | 344±1 | 22.7±0.3 | |
Stability chamber (8°C) | 346±1 | 23.1±0.5 | |
2 Months | Room temperature (25°C) | 347.3±0.5 | 23.7±0.58 |
Refrigerated temperature (40°C) | 349.3±0.5 | 24.2±0.8 | |
Stability chamber (8°C) | 351.2±2.07 | 24.6±1.02 |
CONCLUSION
The biotin-attached magnetite nanoparticles’ consistent size, shape, and surface characteristics specifically increased anticancer activity in MCF-7 breast cancer cells. According to the current study, drug conjugation using magnetite nanoparticles may be an effective method to deliver an anticancer medication to cancer cells, paving the path for utilization in biological applications.
Cite this article:
Nivetha CK, Veintramuthu S, Ponpandianagamony. Fabrication and in vitro Evaluation of Biotin Conjugated Iron Oxide Nanoparticle for Breast Cancer Therapy. Int. J. Pharm. Investigation. 2024;14(4):1235-41.
ACKNOWLEDGEMENT
The facilities required for conducting the study were provided by our respected Principal, Dr. M. Ramanathan and Peelamedu Samanaidu Govindaswamy (PSG) Sons and Charities, and are gratefully acknowledged by the authors.
ABBREVIATIONS
IONP | Iron oxide nanoparticle |
---|---|
EPR | Enhanced permeability and retention |
ER | Estrogen receptor |
AFM | Atomic force microscopy |
XRD | X-ray diffraction |
TGA | Thermal Gravimetric Analysis |
VSM | Vibrating Sample Magnetometer |
NM | Nanometer |
mV | Millivolt |
FT-IR | Fourier Transform Infrared Spectroscopy |
EE | Entrapment efficiency |
IC50 | Half-maximal inhibitory concentration |
NPs | Nanoparticles |
References
- Ali A, Zafar H, Zia M, Ul Haq I, Phull AR, Ali JS, et al. Synthesis, characterization, applications and challenges of iron oxide nanoparticles. Nanotechnol Sci Appl. 2016;9:49-67. [PubMed] | [CrossRef] | [Google Scholar]
- Hosseinkazemi H, Samani S, Soezi M, Moghoofei M, Azhdari MH, Aavani F, et al. Applications of iron oxide nanoparticles against breast cancer. J Nanomate. 2022:1-12. [PubMed] | [CrossRef] | [Google Scholar]
- Rajakaruna TP, Udawatte CP, Chandrajith R, Rajapakse RM. Formulation of iron oxide and oxy-hydroxide nanoparticles from ilmenites and through a low-temperature process. ACS Omega. 2021;6(28):17824-30. [PubMed] | [CrossRef] | [Google Scholar]
- Tassa C, Shaw SY, Weissleder R. Dextran-coated iron oxide nanoparticles a versatile platform for targeted molecular imaging, molecular diagnostics and therapy. Acc Chem Res. 2011;44(10):842-52. [PubMed] | [CrossRef] | [Google Scholar]
- Manna PK, Nickel R, Wroczynskyj Y, Yathindranath V, Li J, Liu S, et al. Simple, hackable, size-selective, amine-functionalized Fe-oxide nanoparticles for biomedical applications. Langmuir. 2018;34(8):2748-57. [PubMed] | [CrossRef] | [Google Scholar]
- Narain R, Gonzales M, Hoffman AS, Stayton PS, Krishnan KM. Synthesis of monodisperse biotinylated p (NIPAAm)-coated iron oxide magnetic nanoparticles and their bioconjugation to streptavidin. Langmuir. 2007;23(11):6299-304. [PubMed] | [CrossRef] | [Google Scholar]
- Hernández-Hernández AA, Aguirre-Álvarez G, Cariño-Cortés R, Mendoza-Huizar LH, Jiménez-Alvarado R. Iron oxide nanoparticles synthesis, functionalization and applications in diagnosis and treatment of cancer. Chem Pap. 2020;74(11):3809-24. [CrossRef] | [Google Scholar]
- Mahmoudi M, Sant S, Wang B, Laurent S, Sen T. Superparamagnetic iron oxide nanoparticles (SPIONs) development, surface modification and applications in chemotherapy. Adv Drug Deliv Rev. 2011;63(1-2):24-46. [PubMed] | [CrossRef] | [Google Scholar]
- Mahmoudi M, Simchi A, Imani M, Milani AS, Stroeve P. Optimal design and characterization of superparamagnetic iron oxide nanoparticles coated with polyvinyl alcohol for targeted delivery and imaging. J Phys Chem B. 2008;112(46):14470-81. [PubMed] | [CrossRef] | [Google Scholar]
- Kumar SR, Priyatharshni S, Babu VN, Mangalaraj D, Viswanathan C, Kannan S, et al. Quercetin conjugated superparamagnetic magnetite nanoparticles for analysis of breast cancer cell lines for chemotherapy applications. J Colloid Interface Sci. 2014;436:234-42. [PubMed] | [CrossRef] | [Google Scholar]
- Simonsen G, Strand M, Norrman J, Øye G. Amino-functionalized iron oxide nanoparticles designed for adsorption of naphthenic acids. Colloids Surf A Physicochem Eng Asp. 2019;568:147-56. [CrossRef] | [Google Scholar]
- Fatima H, Lee DW, Yun HJ, Kim KS. Shape-controlled synthesis of magnetic Fe3O4 nanoparticles with different iron precursors and capping agents. RSC Adv. 2018;8(41):22917-23. [PubMed] | [CrossRef] | [Google Scholar]
- Predescu AM, Matei E, Berbecaru AC, Pantilimon C, Drăgan C, Vidu R, et al. Synthesis and characterization of dextran-coated iron oxide nanoparticles. R Soc Open Sci. 2018;5(3):171525 [PubMed] | [CrossRef] | [Google Scholar]
- Soetaert F, Korangath P, Serantes D, Fiering S, Ivkov R. Cancer therapy with iron oxide nanoparticles Agents of thermal and immune therapies. Adv Drug Deliv Rev. 2020;163-164:65-83. [PubMed] | [CrossRef] | [Google Scholar]
- Gholipour N, Akhlaghi M, Mokhtari Kheirabadi AM, Geramifar P, Beiki D. Development of Ga-68 labeled biotinylated thiosemicarbazone dextran-coated iron oxide nanoparticles as multimodal PET/MRI probe. Int J Biol Macromol. 2020;148:932-41. [PubMed] | [CrossRef] | [Google Scholar]
- Alarifi S, Ali D, Alkahtani S, Alhader MS. Iron oxide nanoparticles induce oxidative stress, DNA damage and caspase activation in the human breast cancer cell line. Biol Trace Elem Res. 2014;159(1-3):416-24. [PubMed] | [CrossRef] | [Google Scholar]